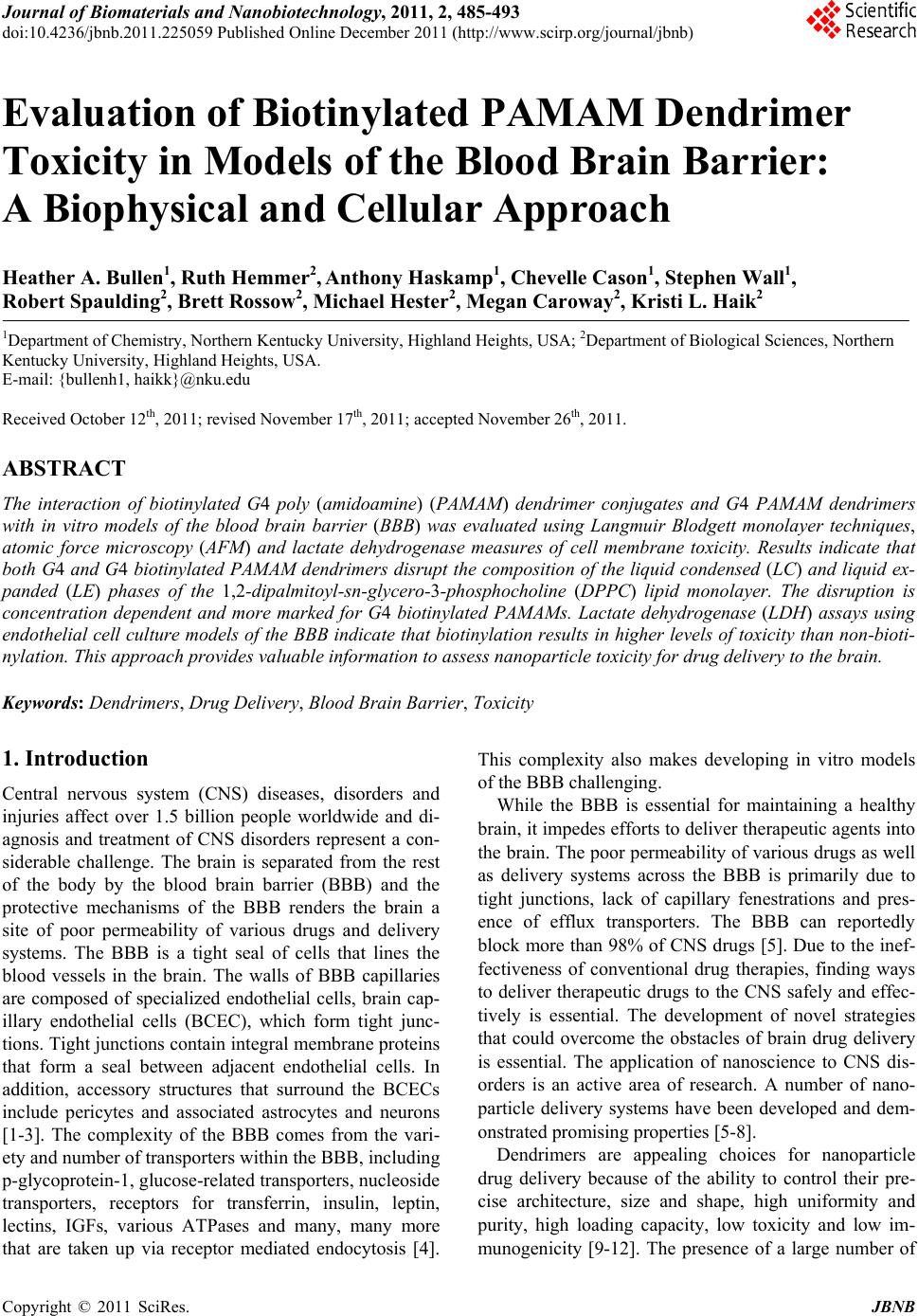 Journal of Biomaterials and Nanobiotechnology, 2011, 2, 485-493 doi:10.4236/jbnb.2011.225059 Published Online December 2011 (http://www.scirp.org/journal/jbnb) Copyright © 2011 SciRes. JBNB 485 Evaluation of Biotinylated PAMAM Dendrimer Toxicity in Models of the Blood Brain Barrier: A Biophysical and Cellular Approach Heather A. Bullen1, Ruth Hemmer2, Anthony Haskamp1, Chevelle Cason1, Stephen Wall1, Robert Spaulding2, Brett Rossow2, Michael Hester2, Megan Caroway2, Kristi L. Haik2 1Department of Chemistry, Northern Kentucky University, Highland Heights, USA; 2Department of Biological Sciences, Northern Kentucky University, Highland Heights, USA. E-mail: {bullenh1, haikk}@nku.edu Received October 12th, 2011; revised November 17th, 2011; accepted November 26th, 2011. ABSTRACT The interaction of biotinylated G4 poly (amidoamine) (PAMAM) dendrimer conjugates and G4 PAMAM dendrimers with in vitro models of the blood brain barrier (BBB) was evaluated using Langmuir Blodgett monolayer techniques, atomic force microscopy (AFM) and lactate dehydrogenase measures of cell membrane toxicity. Results indicate that both G4 and G4 biotinylated PAMAM dendrimers disrupt the composition of the liquid condensed (LC) and liquid ex- panded (LE) phases of the 1,2-dipalmitoyl-sn-glycero-3-phosphocholine (DPPC) lipid monolayer. The disruption is concentration dependent and more marked for G4 biotinylated PAMAMs. Lactate dehydrogenase (LDH) assays using endothelial cell culture models of the BBB indicate that biotinylation results in higher levels of toxicity than non-bioti- nylation. This approach p ro vides valuable information to assess nanoparticle toxicity for drug delivery to the brain. Keywords: Dendrimers, Drug Delivery, Blood Brain Barrier, Toxicity 1. Introduction Central nervous system (CNS) diseases, disorders and injuries affect over 1.5 billion people worldwide and di- agnosis and treatment of CNS disorders represent a con- siderable challenge. The brain is separated from the rest of the body by the blood brain barrier (BBB) and the protective mechanisms of the BBB renders the brain a site of poor permeability of various drugs and delivery systems. The BBB is a tight seal of cells that lines the blood vessels in the brain. The walls of BBB capillaries are composed of specialized endothelial cells, brain cap- illary endothelial cells (BCEC), which form tight junc- tions. Tight junctions contain integral membrane proteins that form a seal between adjacent endothelial cells. In addition, accessory structures that surround the BCECs include pericytes and associated astrocytes and neurons [1-3]. The complexity of the BBB comes from the vari- ety and number of transporters within the BBB, including p-glycoprotein-1, glucose-related transporters, nucleoside transporters, receptors for transferrin, insulin, leptin, lectins, IGFs, various ATPases and many, many more that are taken up via receptor mediated endocytosis [4]. This complexity also makes developing in vitro models of the BBB challenging. While the BBB is essential for maintaining a healthy brain, it impedes efforts to deliver therapeutic agents into the brain. The poor permeability of various drugs as well as delivery systems across the BBB is primarily due to tight junctions, lack of capillary fenestrations and pres- ence of efflux transporters. The BBB can reportedly block more than 98% of CNS drugs [5]. Due to the inef- fectiveness of conventional drug therapies, finding ways to deliver therapeutic drugs to the CNS safely and effec- tively is essential. The development of novel strategies that could overcome the obstacles of brain drug delivery is essential. The application of nanoscience to CNS dis- orders is an active area of research. A number of nano- particle delivery systems have been developed and dem- onstrated promising properties [5-8]. Dendrimers are appealing choices for nanoparticle drug delivery because of the ability to control their pre- cise architecture, size and shape, high uniformity and purity, high loading capacity, low toxicity and low im- munogenicity [9-12]. The presence of a large number of
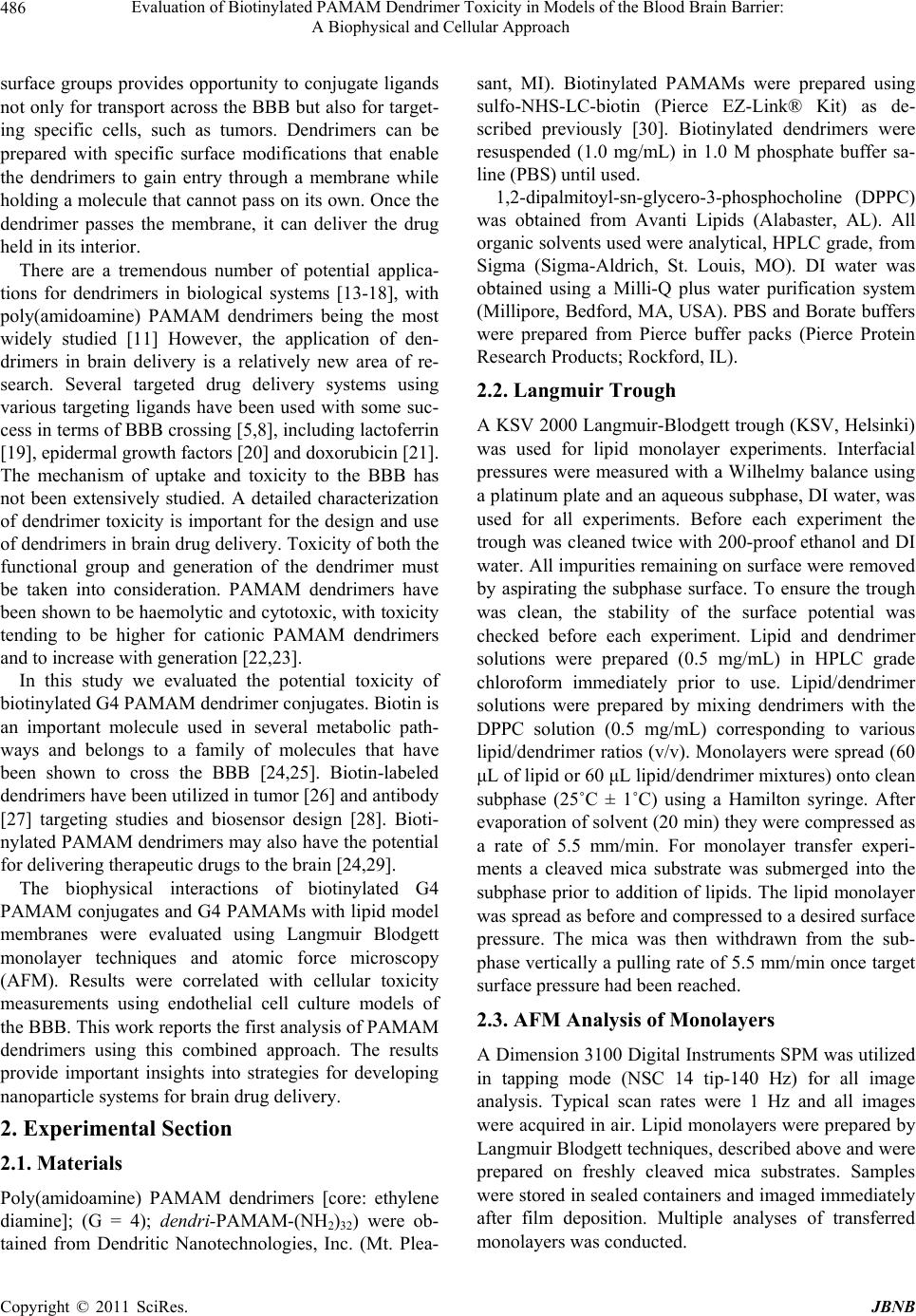 Evaluation of Biotinylated PAMAM Dendrimer Toxicity in Models of the Blood Brain Barrier: 486 A Biophysical and Cellular Approach surface groups provides opportunity to conjugate ligands not only for transport across the BBB but also for target- ing specific cells, such as tumors. Dendrimers can be prepared with specific surface modifications that enable the dendrimers to gain entry through a membrane while holding a molecule that cannot pass on its own. Once the dendrimer passes the membrane, it can deliver the drug held in its interior. There are a tremendous number of potential applica- tions for dendrimers in biological systems [13-18], with poly(amidoamine) PAMAM dendrimers being the most widely studied [11] However, the application of den- drimers in brain delivery is a relatively new area of re- search. Several targeted drug delivery systems using various targeting ligands have been used with some suc- cess in terms of BBB crossing [5,8], including lactoferrin [19], epidermal growth factors [20] and doxorubicin [21]. The mechanism of uptake and toxicity to the BBB has not been extensively studied. A detailed characterization of dendrimer toxicity is important for the design and use of dendrimers in brain drug delivery. Toxicity of both the functional group and generation of the dendrimer must be taken into consideration. PAMAM dendrimers have been shown to be haemolytic and cytotoxic, with toxicity tending to be higher for cationic PAMAM dendrimers and to increase with generation [22,23]. In this study we evaluated the potential toxicity of biotinylated G4 PAMAM dendrimer conjugates. Biotin is an important molecule used in several metabolic path- ways and belongs to a family of molecules that have been shown to cross the BBB [24,25]. Biotin-labeled dendrimers have been utilized in tumor [26] and antibody [27] targeting studies and biosensor design [28]. Bioti- nylated PAMAM dendrimers may also have the potential for delivering therapeutic drugs to the brain [24,29]. The biophysical interactions of biotinylated G4 PAMAM conjugates and G4 PAMAMs with lipid model membranes were evaluated using Langmuir Blodgett monolayer techniques and atomic force microscopy (AFM). Results were correlated with cellular toxicity measurements using endothelial cell culture models of the BBB. This work reports the first analysis of PAMAM dendrimers using this combined approach. The results provide important insights into strategies for developing nanoparticle systems for brain drug delivery. 2. Experimental Section 2.1. Materials Poly(amidoamine) PAMAM dendrimers [core: ethylene diamine]; (G = 4); dendri-PAMAM-(NH2)32) were ob- tained from Dendritic Nanotechnologies, Inc. (Mt. Plea- sant, MI). Biotinylated PAMAMs were prepared using sulfo-NHS-LC-biotin (Pierce EZ-Link® Kit) as de- scribed previously [30]. Biotinylated dendrimers were resuspended (1.0 mg/mL) in 1.0 M phosphate buffer sa- line (PBS) until used. 1,2-dipalmitoyl-sn-glycero-3-phosphocholine (DPPC) was obtained from Avanti Lipids (Alabaster, AL). All organic solvents used were analytical, HPLC grade, from Sigma (Sigma-Aldrich, St. Louis, MO). DI water was obtained using a Milli-Q plus water purification system (Millipore, Bedford, MA, USA). PBS and Borate buffers were prepared from Pierce buffer packs (Pierce Protein Research Products; Rockford, IL). 2.2. Langmuir Trough A KSV 2000 Langmuir-Blodgett trough (KSV, Helsinki) was used for lipid monolayer experiments. Interfacial pressures were measured with a Wilhelmy balance using a platinum plate and an aqueous subphase, DI water, was used for all experiments. Before each experiment the trough was cleaned twice with 200-proof ethanol and DI water. All impurities remaining on surface were removed by aspirating the subphase surface. To ensure the trough was clean, the stability of the surface potential was checked before each experiment. Lipid and dendrimer solutions were prepared (0.5 mg/mL) in HPLC grade chloroform immediately prior to use. Lipid/dendrimer solutions were prepared by mixing dendrimers with the DPPC solution (0.5 mg/mL) corresponding to various lipid/dendrimer ratios (v/v). Monolayers were spread (60 μL of lipid or 60 μL lipid/dendrimer mixtures) onto clean subphase (25˚C ± 1˚C) using a Hamilton syringe. After evaporation of solvent (20 min) they were compressed as a rate of 5.5 mm/min. For monolayer transfer experi- ments a cleaved mica substrate was submerged into the subphase prior to addition of lipids. The lipid monolayer was spread as before and compressed to a desired surface pressure. The mica was then withdrawn from the sub- phase vertically a pulling rate of 5.5 mm/min once target surface pressure had been reached. 2.3. AFM Analysis of Monolayers A Dimension 3100 Digital Instruments SPM was utilized in tapping mode (NSC 14 tip-140 Hz) for all image analysis. Typical scan rates were 1 Hz and all images were acquired in air. Lipid monolayers were prepared by Langmuir Blodgett techniques, described above and were prepared on freshly cleaved mica substrates. Samples were stored in sealed containers and imaged immediately after film deposition. Multiple analyses of transferred monolayers was conducted. Copyright © 2011 SciRes. JBNB
 Evaluation of Biotinylated PAMAM Dendrimer Toxicity in Models of the Blood Brain Barrier: 487 A Biophysical and Cellular Approach 2.4. bEnd.3 Cell Culture A murine brain capillary endothelial cell line, bEnd.3, was purchased from American Type Culture Collection (ATCC, CRL-2299) and cultured as recommended by the supplier. Briefly, bEnd.3 cells were cultured in media containing Dulbecco’s Modified Eagle’s Medium (DMEM; ATCC, 30 - 2002) containing 4 mM L-glutamine, 4500 mg/L glucose, 1 mM sodium pyruvate, 1500 mg/L so- dium bicarbonate and supplemented with penicillin (500 u/mL), streptomycin (100 μg/mL) and 10% fetal bovine serum (FBS) in a humidified 5% CO2, 95% air incubator at 37˚C. At 80% confluency, cells were trypsinized, counted and seeded in 96-well flat-bottomed plates with a density of 1.7 × 105 cells/well. After the cells grew for 2 days, cells were rinsed once with media and cells were then treated with one of the following treatment groups: 3 μL of 1 mg/mL G4 biotinylated dendrimers; 3 μL of 1 mg/mL G4 dendrimers; 3 μL of culture media alone; or 3 μL of 0.05% sodium azide (each added to 197 μL of me- dia). After 24 h of treatment (in humidified 5% CO2, 95% air incubator at 37˚C) the media was collected and used for LDH analysis. Fresh media was added to wells and after 24 h media was collected again for LDH analy- sis. Cell phenotype was confirmed by an immunocyto- chemical stain for von Willebrand factor [31]. 2.5. Lactate Dehydrogenase Assay (LDH) Mouse cerebral endothelial cell death was quantitatively assessed using the Tox-7 LDH based in vitro toxicology assay kit (Sigma). An LDH assay was performed on me- dia collected after 24 and 48 h. LDH values were com- pared between control and treatment conditions using a one-way ANOVA (SPSS) and Bonferroni post-hoc analyses when appropriate. 3. Results and Discussion 3.1. Compression Isotherms Compression isotherms provide information on if PAMAM dendrimers penetrate into the lipid monolayer and influence lipid organization. The surface pressure- area isotherms (π-A isotherms) for DPPC and DPPC with G4 PAMAM and G4 biotinylated PAMAM dendrimers are shown in Figure 1. The isotherm for pure DPPC is similar to that reported in the literature [32,33] and shows the well-known phase transition from liquid-ex- panded (LE) to liquid condensed (LC) phases at around 10 mN/m. Addition of G4 and G4 biotinylated PAMAM dendrimers leads to a slight expansion in the disordered LE phase of the DPPC monolayer and the formation of an indistinct phase transition region. Increasing amounts of G4 and G4 biotinylated PAMAM dendrimers seem to have a greater effect on the disappearance of the DPPC LE-LC phase transition. The PAMAM dendrimers also affected the collapse pressure of the DPPC monolayer (55 mN/m); in general, higher concentrations of PAMAM dendrimers lead to a lower collapse surface pressure. This disruption of lipid monolayer stability is more pro- nounced for G4 biotinylated PAMAM dendrimers (Fig- ure 1(B)). These results indicate that both G4 and G4 bioti- nylated PAMAM dendrimers are initially incorporated into the DPPC monolayer. This incorporation disturbs the organization of the DPPC monolayer, as evident by (A) (B) Figure 1. Surface pressure vs. area isotherm of DPPC lipid monolayers in the presence of different concentrations (v/v) of (A) G4 PAMAM dendrimers and (B) G4 biotinylated PAMAM dendrimers. Copyright © 2011 SciRes. JBNB
 Evaluation of Biotinylated PAMAM Dendrimer Toxicity in Models of the Blood Brain Barrier: 488 A Biophysical and Cellular Approach the shift of the overall isotherm curve to lower molecular areas, loss of the LE-LC phase transition region and de- crease of the surface collapse pressure. The PAMAM dendrimers fluidize the monolayer at low surface pres- sures, causing more lipid molecules to exist in the LE state rather than the LC phase [34-36]. Upon compres- sion an enhanced squeeze out of material into the sub- phase is observed for both DPPC/G4 and DPPC/G4 biotinylated PAMAM dendrimer mixtures, as indicated by the shift of the isotherms to lower molecular areas and the kink evident at ~6 mN/m [34,36]. Previous research suggests that PAMAM dendrimers have an electrostatic nature of binding to lipid membranes [37], implying that primarily the polar head groups of DPPC and the surface amino groups of the G4 PAMAM dendrimers are in- volved in the interaction. This interaction would slightly affect the packing of the acyl chain domain, leading to the area expansion evident in the LE phase [38]. The comparable increase in area expansion in the LE region suggests that the G4 biotinylated PAMAM dendrimers affect the packing density of DPPC in a similar fashion. 3.2. AFM Analysis AFM is a direct imaging tool for visualizing the lipid monolayers to determine the influence of G4 and G4 biotinylated PAMAM dendrimers on changes in the mi- crodomain formation of the LE and LC phases. The AFM analysis of pure DPPC monolayer deposited at 12 mN/m is shown in Figure 2. At this surface pressure, the LE and LC phases coexist, with the bright regions corre- sponding to the LC phase (a more upright configuration) and the dark regions corresponding to the LE phase. The LC phase domains are ~2.2 ± 0.6 µm long and ~124 ± 35 nm wide. These domains are separated by regions of the LE phase (gap is on average ~200 nm). Within the LE Figure 2. AFM height analysis of DPPC lipid monolayer (12 mN/m). phase region smaller islands of LC phase are also evident (~30 - 40 nm in diameter). The influence of G4 and G4 biotinylated PAMAM dendrimers on the DPPC domain structure is shown in Figures 3 and 4. Increasing concentrations of G4 PAM- AM dendrimers reduce the formation of the LC phase domains. At low G4 PAMAM dendrimer concentrations, DPPC:G4, 2:1 (Figure 3(A)), some regions look similar to DPPC alone, with long “stripe-like” LC phase do- mains (~1.0 ± 0.2 µm long; ~68 ± 29 nm wide) separated by ~150 - 200 nm LE phase domains. However other regions show a more significant change in LC phase do- main size, as evident by a more “oval, island-like” struc- tures (~95 ± 20 nm diameter) separated by ~50 nm LE phase domains. Upon increasing concentration of G4 PAMAM dendrimers (DPPC:G4, 1:1), the “oval, is- land-like” LC phase regions become more prevalent within the mono- layer (Figure 3(B)). The LC phase islands (~150 ± 45 nm in diameter) are separated by ~50 nm LE phase domains. In addition a “honeycomb-like” LC phase structure is also evident, exhibiting larger re- gions of LE phase within the monolayer. Under higher concentrations of G4 PAMAM dendrimers (DPPC:G4, 1:2) two different domain structures are apparent, each leading to a further increase in the LE phase composition (Figures 3(C) and 3(D)). Some regions of the monolayer (Figure 3(C)) show LC phase islands that are quite var- ied in shape (roughly ~500 nm diameter) separated by LE phase regions (~300 - 400 nm gaps), which make up ~68% of the monolayer composition. Other regions show further in- creases in LE phase composition (Figure 3(D)) with the LC phase existing as lines (~720 ± 180 nm long; ~31 ± 4 nm wide) separated by LE phase regions (~300- 350 nm gaps), which make up ~80% of the monolayer composition. Low concentrations of G4 biotinylated PAMAM den- drimers, DPPC:G4bio, 2:1 (Figure 4(A)), cause an ag- gregation of the LC phase to form larger stripes com- pared to those found in DPPC alone. The LC and LE phase domains coexist as alternating stripes (>3 µm long; ~273 ± 86 nm wide); within the LE regions, small islands (~35 - 50 nm diameter) of the LC phase also exist. Upon increasing concentration of G4 biotinylated PAMAM dendrimers (DPPC:G4bio, 1:1), two different domain structures are evident (Figures 4(B) and 4(C)), both showing an increase in the LE phase composition of the monolayer. Figure 4(B) shows LC phase stripe domains (~800 ± 150 nm long, ~210 ± 80 nm wide) separated by LE phase regions (~400 nm - 1.5 µm gaps), which make up ~80% of the monolayer composition. Figure 4(C) shows LC islands domains that vary in shape (roughly 1 µm in diameter) separated byhase regions (~350 nm LE p Copyright © 2011 SciRes. JBNB
 Evaluation of Biotinylated PAMAM Dendrimer Toxicity in Models of the Blood Brain Barrier: A Biophysical and Cellular Approach Copyright © 2011 SciRes. JBNB 489 Figure 3. AFM height analysis of DPPC lipid monolayers (12 mN/m) in the presence of different concentrations (v/v) of G4 PAMAM dendrimers: (A) DPPC:G4, 2:1; (B) DPPC:G4, 1 : 1; (C) DPPC:G4, 1:2; (D) DPPC:G4, 1:2. - 600 nm gaps), which makeup ~65% of the monolayer composition. Under higher concentrations of G4 bioti- nylated PAMAM dendrimers (DPPC:G4bio, 1:2) a fur- ther increase in the LE phase region is evident (Figure 4D). The LC phase exists as lines (~716 ± 210 nm long; ~24 ± 6 nm wide) separated by LE phase regions (~250 nm gaps). Larger regions of LE phase (1 - 2 μm) are also evident, with the LE phase making up ~89% of the mono- layer composition. AFM analysis reveals that both G4 and G4 bioti- nylated PAMAM dendrimer conjugates disrupt the com- position of the LC and LE phases of DPPC. The disrupt- tion is concentration dependent and more marked for G4 biotinylated PAMAM dendrimers. These findings are in agreement with compression isotherm measurements, which showed a change in the LE-LC phase transition and decrease in collapse pressure, reflecting a clear de- stabilization of the DPPC packing upon addition of the PAMAM dendrimers. AFM analysis showed no evidence of PAMAM dendrimers within the monolayers. This is also in agreement with compression isotherm analysis which indicated that there was a squeeze out of material from the monolayer upon compression to higher surface pressures. The occupation of lower molecular areas for the isotherm suggests that in addition to the PAMAM dendrimers, lipids may also have been expelled from the monolayer. One possible mechanism could be that strong lipid-polymer interactions cause the DPPC molecules to surround the dendrimers, forming vesicles in solution that escape into the subphase [39]. 3.3. Cell Toxicity The bEnd.3 immortalized endothelial cell line was cho- sen as a basic model of the BBB. Endothelial cells make up a majority of the BBB, which controls cerebral ho- meostasis and prevents toxins and other chemicals in the blood stream from entering the brain. Therefore, it is vital to understand how nanoparticles affect these cells. The endothelial phenotype of the bEnd.3 cell line was confirmed by the observed expression of von Willebrand factor and uptake of fluorescently labeled low density lipoprotein (LDL) [31]. To obtain a specific measure of toxicity, the LDH as- say was used for spectrophotometric measurement of
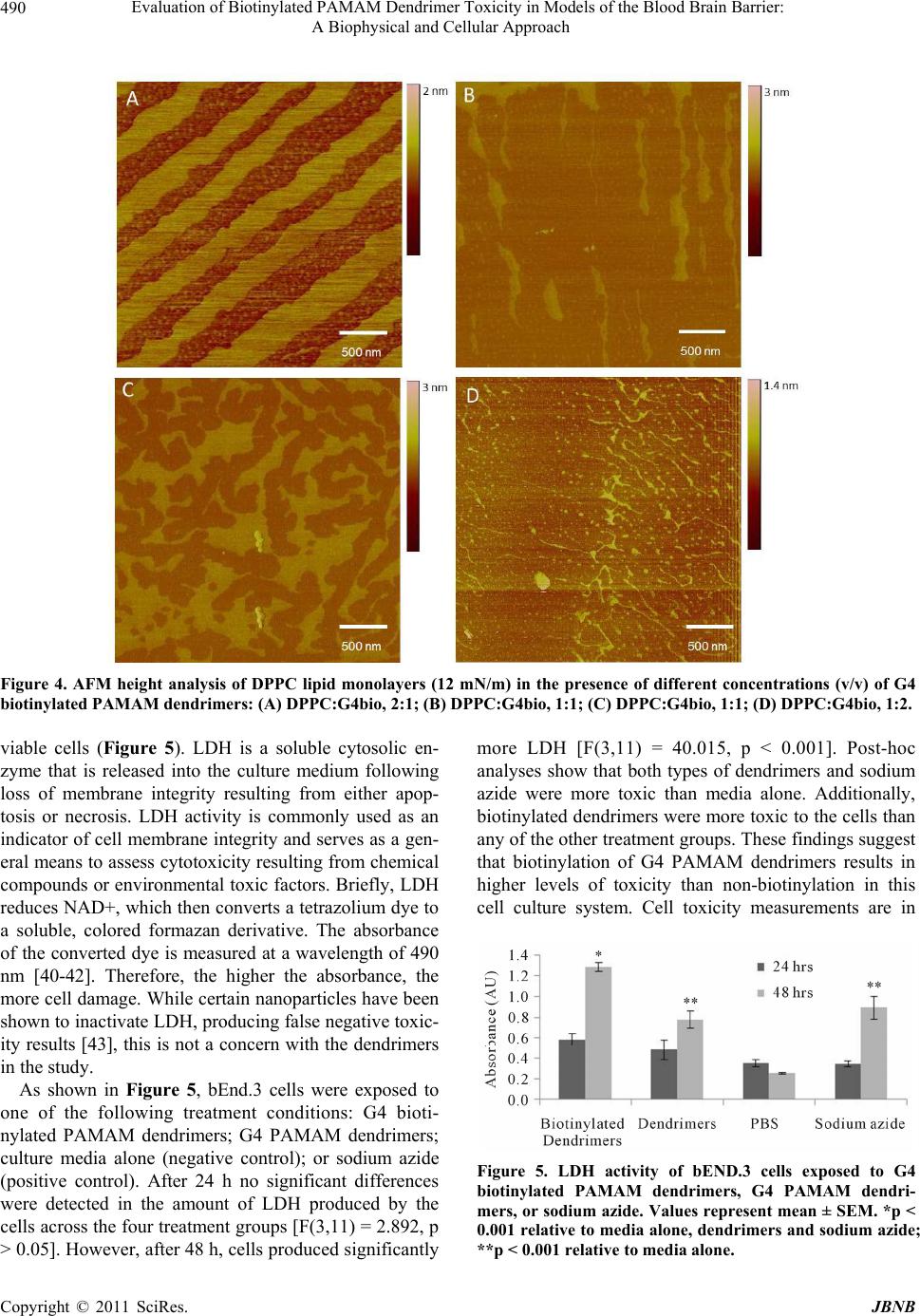 Evaluation of Biotinylated PAMAM Dendrimer Toxicity in Models of the Blood Brain Barrier: 490 A Biophysical and Cellular Approach Figure 4. AFM height analysis of DPPC lipid monolayers (12 mN/m) in the presence of different concentrations (v/v) of G4 biotinylated PAMAM dendrimers: (A) DPPC:G4bio, 2:1; (B) DPPC:G4bio, 1:1; (C) DPPC:G4bio, 1:1; (D) DPPC:G4bio, 1:2. viable cells (Figure 5). LDH is a soluble cytosolic en- zyme that is released into the culture medium following loss of membrane integrity resulting from either apop- tosis or necrosis. LDH activity is commonly used as an indicator of cell membrane integrity and serves as a gen- eral means to assess cytotoxicity resulting from chemical compounds or environmental toxic factors. Briefly, LDH reduces NAD+, which then converts a tetrazolium dye to a soluble, colored formazan derivative. The absorbance of the converted dye is measured at a wavelength of 490 nm [40-42]. Therefore, the higher the absorbance, the more cell damage. While certain nanoparticles have been shown to inactivate LDH, producing false negative toxic- ity results [43], this is not a concern with the dendrimers in the study. As shown in Figure 5, bEnd.3 cells were exposed to one of the following treatment conditions: G4 bioti- nylated PAMAM dendrimers; G4 PAMAM dendrimers; culture media alone (negative control); or sodium azide (positive control). After 24 h no significant differences were detected in the amount of LDH produced by the cells across the four treatment groups [F(3,11) = 2.892, p > 0.05]. However, after 48 h, cells produced significantly more LDH [F(3,11) = 40.015, p < 0.001]. Post-hoc analyses show that both types of dendrimers and sodium azide were more toxic than media alone. Additionally, biotinylated dendrimers were more toxic to the cells than any of the other treatment groups. These findings suggest that biotinylation of G4 PAMAM dendrimers results in higher levels of toxicity than non-biotinylation in this cell culture system. Cell toxicity measurements are in Figure 5. LDH activity of bEND.3 cells exposed to G4 biotinylated PAMAM dendrimers, G4 PAMAM dendri- mers, or sodium azide. Values represent mean ± SEM. *p < 0.001 relative to media alone, dendrimers and sodium azide; **p < 0.001 relative to media alone. Copyright © 2011 SciRes. JBNB
 Evaluation of Biotinylated PAMAM Dendrimer Toxicity in Models of the Blood Brain Barrier: 491 A Biophysical and Cellular Approach agreement with Langmuir compression isotherms and AFM analyses. Although it is important to note that the dosage of dendrimers may impact toxicity measurements [44]. 4. Conclusions The findings presented here show the potential toxicity of G4 and G4 biotinylated PAMAM dendrimers and how biophysical measurements with model lipid systems can be correlated with cell toxicity analysis to provide infor- mation on nanoparticle toxicity. At this time the exact factors that lead to the increased toxicity measured for biotinylated PAMAM dendrimers are unknown. How- ever, controlling the degree of surface functionalization could potentially reduce dendrimer toxicity [22] (currently ~17% surface coverage) [30]. It is possible that biotiny- lated PAMAM dendrimers may prove to be more toxic compared to PAMAM dendrimers alone, in part, due to their potential mechanism of uptake across the BBB, as biotin has shown to cross the BBB through carrier me- diated endocytosis [24,25]. Therefore, there may be more biotinylated PAMAM dendrimer conjugates getting into cells compared to non-biotinylated PAMAM dendrimers, leading to more cell death. Although it is important to note that the dosage of dendrimers may impact toxicity measurements [44]. 5. Acknowledgements This project has been funded by the Merck Institute for Science Education, Kentucky NSF EPSCoR, Northern Kentucky University, Center for Integrated Natural Sci- ence and Mathematics and the NKU Research Founda- tion. This work was also supported by a grant from the Kentucky Biomedical Research Infrastructure Network (P20 RR016481-08) and the National Science Founda- tion (DMR-0526686, CHE-0619342). REFERENCES [1] R. Dermietzel and D. Krause, “Molecular Anatomy of the Blood-Brain Barrier as Defined by Immunocytochemis- try,” International Review Cytology, Vol. 127, No. 1991, pp. 57-109. [2] T. S. Reese and M. J. Karnovsky, “Fine Structural Local- ization of a Blood-Brain Barrier to Exogenous Peroxi- dase,” The Journal of Cell Biology, Vol. 34, No. 1, 1967, pp. 207-217. doi:10.1083/jcb.34.1.207 [3] G. Savettieri, I. Di Liegro, C. Catania, L. Licata, G. L. Pitarresi, S. D’Agostino, G. Schiera, V. De Caro, G. Giandalia, L. I. Giannola and A. Cestelli, “Neurons and ECM Regulate Occludin Localization in Brain Endothe- lial Cells,” NeuroReport, Vol. 11, No. 5, 2000, pp. 1081-1084. doi:10.1097/00001756-200004070-00035 [4] K. Mertsch and M. Jochen, “Blood-Brain Barrier Penetra- tion and Drug Development from an Industrial Point of View,” Current Medicinal Chemistry: Central Nervous System Agents, Vol. 2, No. 3, 2002, pp. 187-201. doi:10.2174/1568015023358067 [5] H. Yang, “Nanoparticle-Mediated Brain-Specific Drug Delivery, Imaging and Diagnosis,” Pharmaceutical Re- search, Vol. 27, No. 9, 2010, pp. 1759-1771. doi:10.1007/s11095-010-0141-7 [6] S. Bhaskar, F. Tian, T. Stoeger, W. Kreyling, J. de la Fuente, V. Grazú, P. Borm, G. Estrada, V. Ntziachristos and D. Razansky, “Multifunctional Nanocarriers for Di- agnostics, Drug Delivery and Targeted Treatment across Blood-Brain Barrier: Perspectives on Tracking and Neuro- imaging,” Particle and Fibre Toxicology, Vol. 7, No. 3, 2010, pp. 1-25. [7] A. Agarwal, N. Lariya, G. Saraogi, N. Dubey, H. Agrawal and G. P. Agrawal, “Nanoparticles as Novel Carrier for Brain Delivery: A Review,” Current Pharmaceutical De- sign, Vol. 15, No. 8, 2009, pp. 917-925. doi:10.2174/138161209787582057 [8] J. L. Gilmore, Y. Xiang, Q. Lingdong and A. Kabanov, “Novel Nanomaterials for Clinical Neuroscience,” Jour- nal of NeuroImmune Pharmacology, Vol. 3, No. 2, 2008, pp. 83-94. doi:10.1007/s11481-007-9099-6 [9] G. M. Dykes, “Dendrimers: A Review of Their Appeal and Applications,” Journal of Chemical Technology and Biotechnology, Vol. 76, No. 9, 2001, pp. 903-918. doi:10.1002/jctb.464 [10] D. A. Tomalia, “Birth of a New Macromolecular Archi- tecture: Dendrimers as Quantized Building Blocks for Nanoscale Synthetic Organic Chemistry,” Progress in Polymer Science, Vol. 30, No. 3-4, 2005, pp. 294-324. doi:10.1016/j.progpolymsci.2005.01.007 [11] D. A. Tomalia, “The Emergence of a New Macromo- lecular Architecture: The Dendritic State,” In: J. E. Mark, Ed., Physical Properties of Polymers Handbook, Springer, New York, 2007, pp. 671-692. doi:10.1007/978-0-387-69002-5_42 [12] D. A. Tomalia, S. A. Henderson and M. S. Diallo, “Den- drimers—An Enabling Synthetic Science to Controlled Organic Nanostructures,” In: W. A. I. Goddard, D. W. Brenner, S. E. Lyshevski and G. J. Iafrate, Eds., Hand- book of Nanoscience, Engineering and Technology, CRC Press, Boca Raton, 2007, pp. 24.1-24.47. doi:10.1201/9781420007848.ch24 [13] D. Astruc, E. Boisselier and C. Ornelas, “Dendrimers Designed for Functions: From Physical, Photophysical and Supramolecular Properties to Applications in Sensing, Catalysis, Molecular Electronics, Photonics and Nano- medicine,” Chemical Revi ews, Vol. 110, No. 4, 2010, pp. 1857-1959.doi:10.1021/cr900327d [14] V. Gajbhiye, V. K. Palanirajan, R. K. Tekade and N. K. Jain, “Dendrimers as Therapeutic Agents: A Systematic Review,” Journal of Pharmacy and Pharmacology, Vol. 61, No. 8, 2010, pp. 989-1003. doi:10.1211/jpp.61.08.0002 [15] M. A. Mintzer and M. W. Grinstaff, “Biomedical Appli- Copyright © 2011 SciRes. JBNB
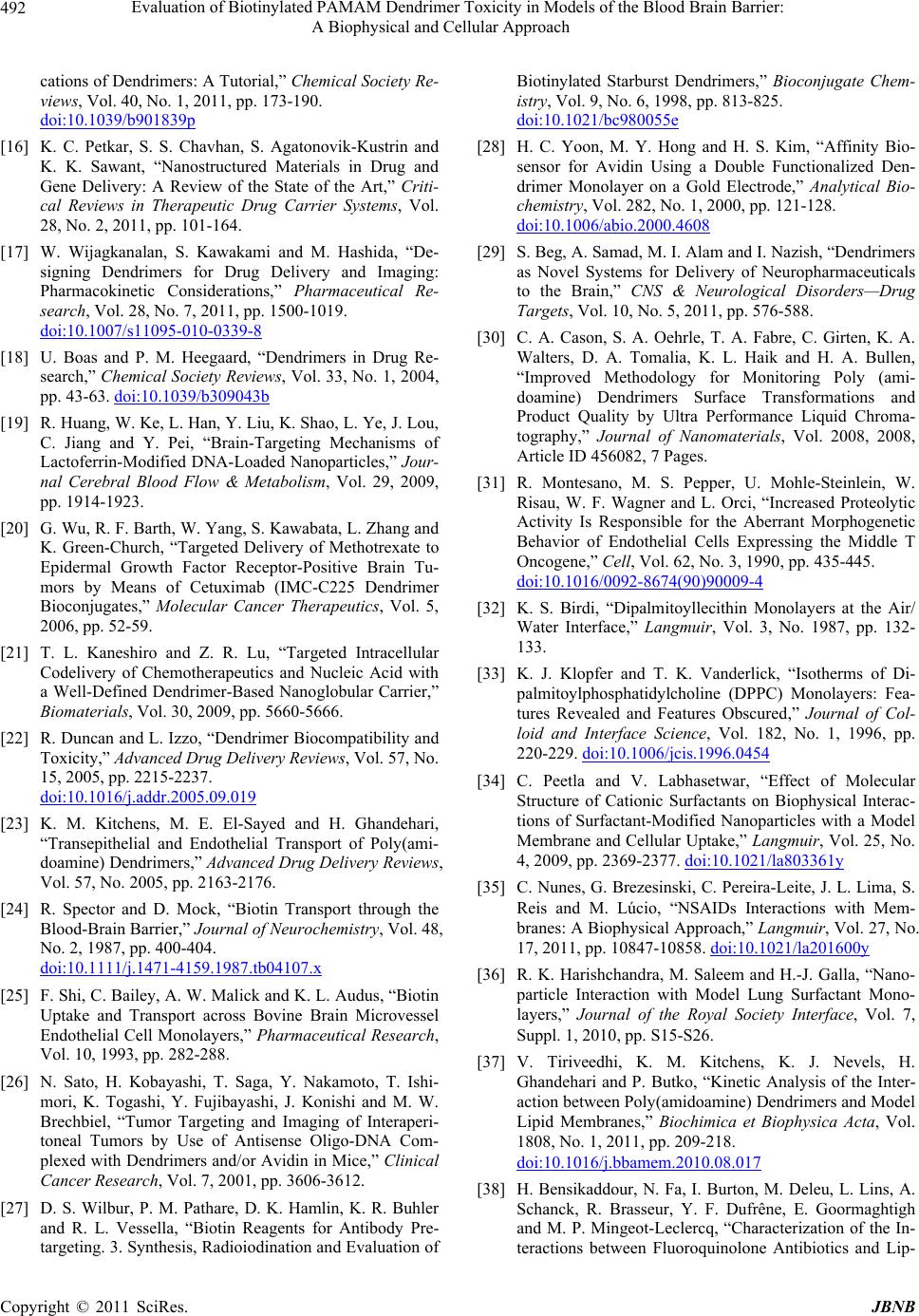 Evaluation of Biotinylated PAMAM Dendrimer Toxicity in Models of the Blood Brain Barrier: 492 A Biophysical and Cellular Approach cations of Dendrimers: A Tutorial,” Chemical Society Re- views, Vol. 40, No. 1, 2011, pp. 173-190. doi:10.1039/b901839p [16] K. C. Petkar, S. S. Chavhan, S. Agatonovik-Kustrin and K. K. Sawant, “Nanostructured Materials in Drug and Gene Delivery: A Review of the State of the Art,” Criti- cal Reviews in Therapeutic Drug Carrier Systems, Vol. 28, No. 2, 2011, pp. 101-164. [17] W. Wijagkanalan, S. Kawakami and M. Hashida, “De- signing Dendrimers for Drug Delivery and Imaging: Pharmacokinetic Considerations,” Pharmaceutical Re- search, Vol. 28, No. 7, 2011, pp. 1500-1019. doi:10.1007/s11095-010-0339-8 [18] U. Boas and P. M. Heegaard, “Dendrimers in Drug Re- search,” Chemical Society Reviews, Vol. 33, No. 1, 2004, pp. 43-63. doi:10.1039/b309043b [19] R. Huang, W. Ke, L. Han, Y. Liu, K. Shao, L. Ye, J. Lou, C. Jiang and Y. Pei, “Brain-Targeting Mechanisms of Lactoferrin-Modified DNA-Loaded Nanoparticles,” Jour- nal Cerebral Blood Flow & Metabolism, Vol. 29, 2009, pp. 1914-1923. [20] G. Wu, R. F. Barth, W. Yang, S. Kawabata, L. Zhang and K. Green-Church, “Targeted Delivery of Methotrexate to Epidermal Growth Factor Receptor-Positive Brain Tu- mors by Means of Cetuximab (IMC-C225 Dendrimer Bioconjugates,” Molecular Cancer Therapeutics, Vol. 5, 2006, pp. 52-59. [21] T. L. Kaneshiro and Z. R. Lu, “Targeted Intracellular Codelivery of Chemotherapeutics and Nucleic Acid with a Well-Defined Dendrimer-Based Nanoglobular Carrier,” Biomaterials, Vol. 30, 2009, pp. 5660-5666. [22] R. Duncan and L. Izzo, “Dendrimer Biocompatibility and Toxicity,” Advanced Drug Delivery Reviews, Vol. 57, No. 15, 2005, pp. 2215-2237. doi:10.1016/j.addr.2005.09.019 [23] K. M. Kitchens, M. E. El-Sayed and H. Ghandehari, “Transepithelial and Endothelial Transport of Poly(ami- doamine) Dendrimers,” Advanced Drug Delivery Reviews, Vol. 57, No. 2005, pp. 2163-2176. [24] R. Spector and D. Mock, “Biotin Transport through the Blood-Brain Barrier,” Journal of Neurochemistry, Vol. 48, No. 2, 1987, pp. 400-404. doi:10.1111/j.1471-4159.1987.tb04107.x [25] F. Shi, C. Bailey, A. W. Malick and K. L. Audus, “Biotin Uptake and Transport across Bovine Brain Microvessel Endothelial Cell Monolayers,” Pharmaceutical Research, Vol. 10, 1993, pp. 282-288. [26] N. Sato, H. Kobayashi, T. Saga, Y. Nakamoto, T. Ishi- mori, K. Togashi, Y. Fujibayashi, J. Konishi and M. W. Brechbiel, “Tumor Targeting and Imaging of Interaperi- toneal Tumors by Use of Antisense Oligo-DNA Com- plexed with Dendrimers and/or Avidin in Mice,” Clinical Cancer Research, Vol. 7, 2001, pp. 3606-3612. [27] D. S. Wilbur, P. M. Pathare, D. K. Hamlin, K. R. Buhler and R. L. Vessella, “Biotin Reagents for Antibody Pre- targeting. 3. Synthesis, Radioiodination and Evaluation of Biotinylated Starburst Dendrimers,” Bioconjugate Chem- istry, Vol. 9, No. 6, 1998, pp. 813-825. doi:10.1021/bc980055e [28] H. C. Yoon, M. Y. Hong and H. S. Kim, “Affinity Bio- sensor for Avidin Using a Double Functionalized Den- drimer Monolayer on a Gold Electrode,” Analytical Bio- chemistry, Vol. 282, No. 1, 2000, pp. 121-128. doi:10.1006/abio.2000.4608 [29] S. Beg, A. Samad, M. I. Alam and I. Nazish, “Dendrimers as Novel Systems for Delivery of Neuropharmaceuticals to the Brain,” CNS & Neurological Disorders—Drug Targets, Vol. 10, No. 5, 2011, pp. 576-588. [30] C. A. Cason, S. A. Oehrle, T. A. Fabre, C. Girten, K. A. Walters, D. A. Tomalia, K. L. Haik and H. A. Bullen, “Improved Methodology for Monitoring Poly (ami- doamine) Dendrimers Surface Transformations and Product Quality by Ultra Performance Liquid Chroma- tography,” Journal of Nanomaterials, Vol. 2008, 2008, Article ID 456082, 7 Pages. [31] R. Montesano, M. S. Pepper, U. Mohle-Steinlein, W. Risau, W. F. Wagner and L. Orci, “Increased Proteolytic Activity Is Responsible for the Aberrant Morphogenetic Behavior of Endothelial Cells Expressing the Middle T Oncogene,” Cell, Vol. 62, No. 3, 1990, pp. 435-445. doi:10.1016/0092-8674(90)90009-4 [32] K. S. Birdi, “Dipalmitoyllecithin Monolayers at the Air/ Water Interface,” Langmuir, Vol. 3, No. 1987, pp. 132- 133. [33] K. J. Klopfer and T. K. Vanderlick, “Isotherms of Di- palmitoylphosphatidylcholine (DPPC) Monolayers: Fea- tures Revealed and Features Obscured,” Journal of Col- loid and Interface Science, Vol. 182, No. 1, 1996, pp. 220-229. doi:10.1006/jcis.1996.0454 [34] C. Peetla and V. Labhasetwar, “Effect of Molecular Structure of Cationic Surfactants on Biophysical Interac- tions of Surfactant-Modified Nanoparticles with a Model Membrane and Cellular Uptake,” Langmuir, Vol. 25, No. 4, 2009, pp. 2369-2377. doi:10.1021/la803361y [35] C. Nunes, G. Brezesinski, C. Pereira-Leite, J. L. Lima, S. Reis and M. Lúcio, “NSAIDs Interactions with Mem- branes: A Biophysical Approach,” Langmuir, Vol. 27, No. 17, 2011, pp. 10847-10858. doi:10.1021/la201600y [36] R. K. Harishchandra, M. Saleem and H.-J. Galla, “Nano- particle Interaction with Model Lung Surfactant Mono- layers,” Journal of the Royal Society Interface, Vol. 7, Suppl. 1, 2010, pp. S15-S26. [37] V. Tiriveedhi, K. M. Kitchens, K. J. Nevels, H. Ghandehari and P. Butko, “Kinetic Analysis of the Inter- action between Poly(amidoamine) Dendrimers and Model Lipid Membranes,” Biochimica et Biophysica Acta, Vol. 1808, No. 1, 2011, pp. 209-218. doi:10.1016/j.bbamem.2010.08.017 [38] H. Bensikaddour, N. Fa, I. Burton, M. Deleu, L. Lins, A. Schanck, R. Brasseur, Y. F. Dufrêne, E. Goormaghtigh and M. P. Mingeot-Leclercq, “Characterization of the In- teractions between Fluoroquinolone Antibiotics and Lip- Copyright © 2011 SciRes. JBNB
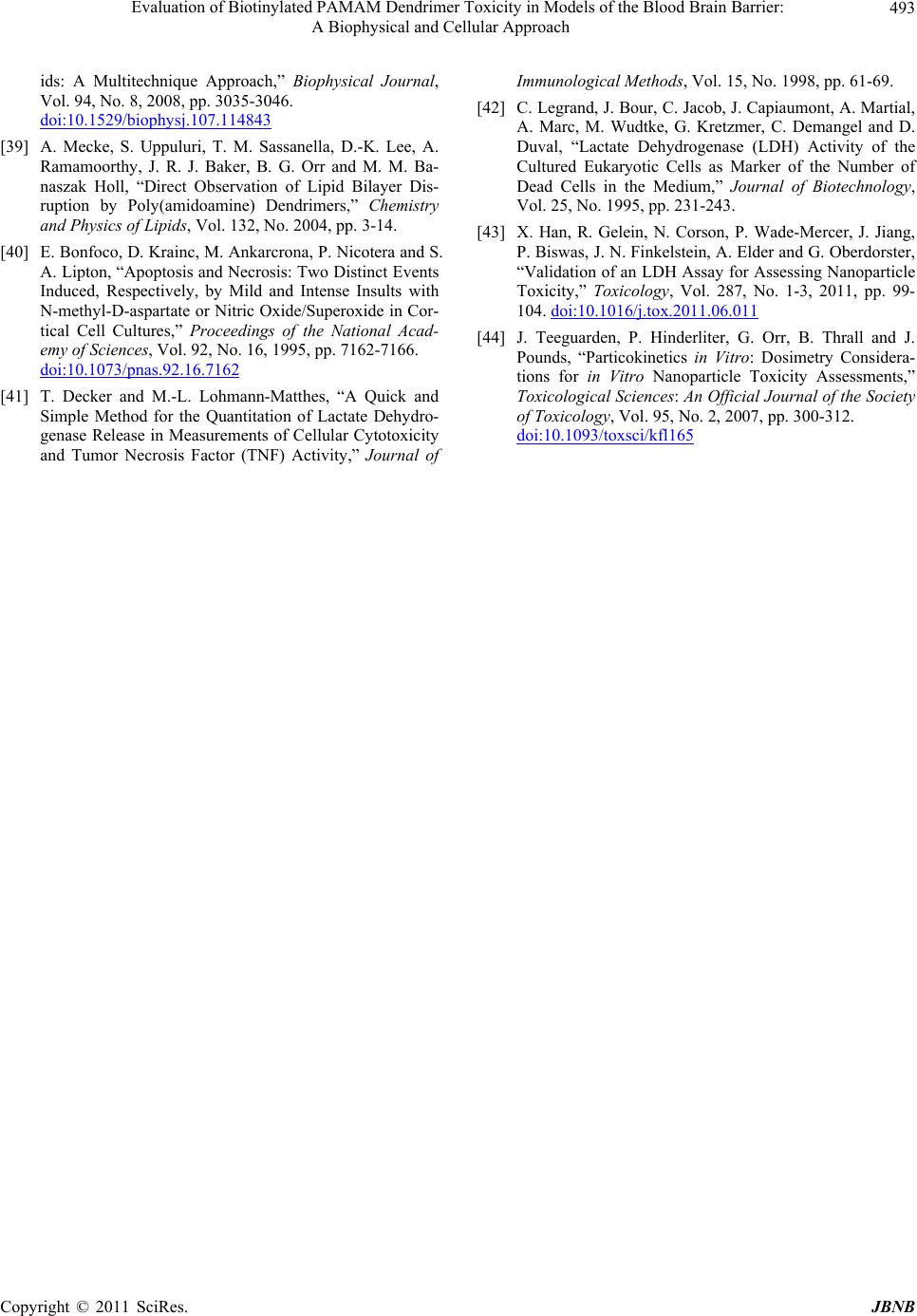 Evaluation of Biotinylated PAMAM Dendrimer Toxicity in Models of the Blood Brain Barrier: A Biophysical and Cellular Approach Copyright © 2011 SciRes. JBNB 493 ids: A Multitechnique Approach,” Biophysical Journal, Vol. 94, No. 8, 2008, pp. 3035-3046. doi:10.1529/biophysj.107.114843 [39] A. Mecke, S. Uppuluri, T. M. Sassanella, D.-K. Lee, A. Ramamoorthy, J. R. J. Baker, B. G. Orr and M. M. Ba- naszak Holl, “Direct Observation of Lipid Bilayer Dis- ruption by Poly(amidoamine) Dendrimers,” Chemistry and Physics of Lipids, Vol. 132, No. 2004, pp. 3-14. [40] E. Bonfoco, D. Krainc, M. Ankarcrona, P. Nicotera and S. A. Lipton, “Apoptosis and Necrosis: Two Distinct Events Induced, Respectively, by Mild and Intense Insults with N-methyl-D-aspartate or Nitric Oxide/Superoxide in Cor- tical Cell Cultures,” Proceedings of the National Acad- emy of Sciences, Vol. 92, No. 16, 1995, pp. 7162-7166. doi:10.1073/pnas.92.16.7162 [41] T. Decker and M.-L. Lohmann-Matthes, “A Quick and Simple Method for the Quantitation of Lactate Dehydro- genase Release in Measurements of Cellular Cytotoxicity and Tumor Necrosis Factor (TNF) Activity,” Journal of Immunological Metho d s, Vol. 15, No. 1998, pp. 61-69. [42] C. Legrand, J. Bour, C. Jacob, J. Capiaumont, A. Martial, A. Marc, M. Wudtke, G. Kretzmer, C. Demangel and D. Duval, “Lactate Dehydrogenase (LDH) Activity of the Cultured Eukaryotic Cells as Marker of the Number of Dead Cells in the Medium,” Journal of Biotechnology, Vol. 25, No. 1995, pp. 231-243. [43] X. Han, R. Gelein, N. Corson, P. Wade-Mercer, J. Jiang, P. Biswas, J. N. Finkelstein, A. Elder and G. Oberdorster, “Validation of an LDH Assay for Assessing Nanoparticle Toxicity,” Toxicology, Vol. 287, No. 1-3, 2011, pp. 99- 104. doi:10.1016/j.tox.2011.06.011 [44] J. Teeguarden, P. Hinderliter, G. Orr, B. Thrall and J. Pounds, “Particokinetics in Vitro: Dosimetry Considera- tions for in Vitro Nanoparticle Toxicity Assessments,” Toxicological Sciences: An Official Journal of the Society of Toxicology, Vol. 95, No. 2, 2007, pp. 300-312. doi:10.1093/toxsci/kfl165
|