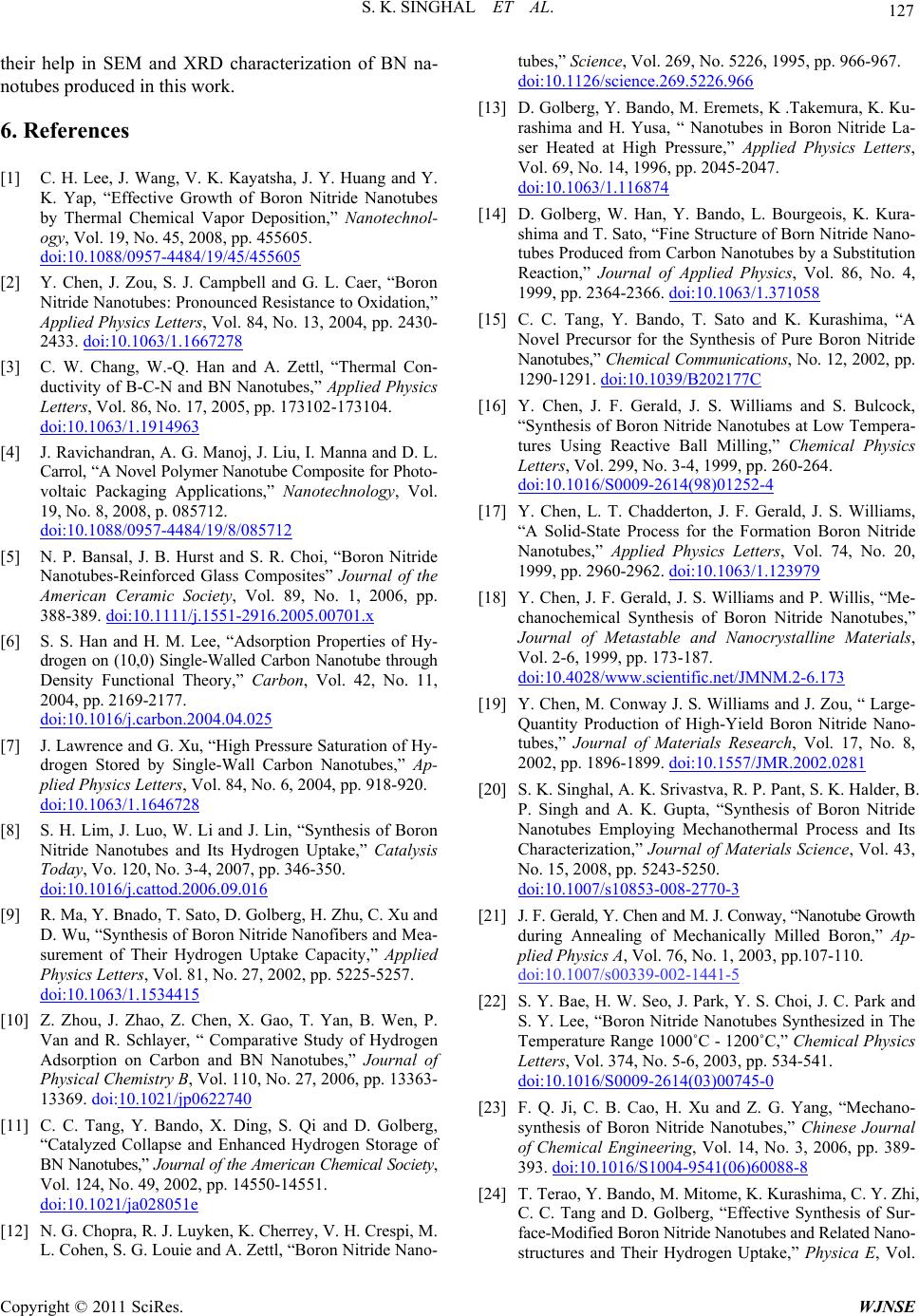
S. K. SINGHAL ET AL. 127
] C. H. Lee, J. Wang, V. K. Kayatsha, J. Y. Huang and Y.
of Boron Nitride Nanotubes
Deposition,” Nanotechnol-
their help in SEM and XRD characterization of BN na-
notubes produced in this work.
6. References
[1
K. Yap, “Effective Growth
by Thermal Chemical Vapor
ogy, Vol. 19, No. 45, 2008, pp. 455605.
doi:10.1088/0957-4484/19/45/455605
[2] Y. Chen, J. Zou, S. J. Campbell and G. L. Caer, “Boron
Nitride Nanotubes: Pronounced Resistance
Applied Physics Letters, Vol. 84, No. 1
to Oxidation,”
3, 2004, pp. 2430-
2433. doi:10.1063/1.1667278
[3] C. W. Chang, W.-Q. Han and A. Zettl, “Thermal Con-
ductivity of B-C-N and BN Nanotubes,” Applied Physics
Letters, Vol. 86, No. 17, 2005, pp. 173102-173104.
doi:10.1063/1.1914963
[4] J. Ravichandran, A. G. Manoj, J. Liu, I. Manna and D. L.
Carrol, “A Novel Polymer Nanotube Composite for Ph
voltaic Packaging Appl
oto-
ications,” Nanotechnology, Vol.
19, No. 8, 2008, p. 085712.
doi:10.1088/0957-4484/19/8/085712
[5] N. P. Bansal, J. B. Hurst and S. R. Choi, “Boron Nitride
Nanotubes-Reinforced Glass
American Ceramic Society, Vol. 89
Composites” Journal of th
, No. 1, 2006, pp.
e
388-389. doi:10.1111/j.1551-2916.2005.00701.x
[6] S. S. Han and H. M. Lee, “Adsorption Properties of Hy-
drogen on (10,0) Single-Walled Carbon Nanotube through
Density Functional Theory,” Carbon, Vol. 42, No. 11,
2004, pp. 2169-2177.
doi:10.1016/j.carbon.2004.04.025
[7] J. Lawrence and G. Xu, “High Pressure Saturation of Hy-
drogen Stored by Single-Wall Carbon Nanotubes,” Ap-
plied Physics Letters, Vol. 84, No. 6, 2004, pp. 918-920.
doi:10.1063/1.1646728
[8] S. H. Lim, J. Luo, W. Li and J. Lin, “Synthesis of Boron
Nitride Nanotubes and Its Hydrogen Uptake,” Catalysis
Today, Vo. 120, No. 3-4, 2007, pp. 346-350.
doi:10.1016/j.cattod.2006.09.016
[9] R. Ma, Y. Bnado, T. Sato, D. Golberg, H. Zhu, C. Xu and
D. Wu, “Synthesis of Boron Nitride Nanofibers
surement of Their Hydrogen Uptake C
and Mea-
apacity,” Applied
Physics Letters, Vol. 81, No. 27, 2002, pp. 5225-5257.
doi:10.1063/1.1534415
[10] Z. Zhou, J. Zhao, Z. Chen, X. Gao, T. Yan, B. Wen, P.
Van and R. Schlayer, “ Comparative Study of Hydrogen
Adsorption on Carbon
and BN Nanotubes,” Journal of
Physical Chemistry B, Vol. 110, No. 27, 2006, pp. 13363-
13369. doi:10.1021/jp0622740
[11] C. C. Tang, Y. Bando, X. Ding, S. Qi and D. Golberg,
“Catalyzed Collapse and Enhanced Hydrogen Storage of
BN Nanotubes,” Journal of the American Chemical Society,
Vol. 124, No. 49, 2002, pp. 14550-14551.
doi:10.1021/ja028051e
[12] N. G. Chopra, R. J. Luyken, K. Cherrey, V. H. Crespi, M.
L. Cohen, S. G. Louie and A. Zettl, “Boron N
tubes,” Science, Vol. 269
itride Nano-
, No. 5226, 1995, pp. 966-967.
doi:10.1126/science.269.5226.966
[13] D. Golberg, Y. Bando, M. Eremets, K .Takemura, K. Ku-
rashima and H. Yusa, “ Nanotubes in Boron Nitride La-
ser Heated at High Pressure,” Applied Physics Letters,
Vol. 69, No. 14, 1996, pp. 2045-2047.
doi:10.1063/1.116874
[14] D. Golberg, W. Han, Y. Bando, L. Bourgeois, K. Kura-
shima and T. Sato, “Fine Structure of Bo
tubes Produced from C
rn Nitride Nano-
arbon Nanotubes by a Substitution
Reaction,” Journal of Applied Physics, Vol. 86, No. 4,
1999, pp. 2364-2366. doi:10.1063/1.371058
[15] C. C. Tang, Y. Bando, T. Sato and K. Kurashima, “A
Novel Precursor for the Synthesis of Pure Boron Nitride
Nanotubes,” Chemical Communications, No. 12, 2002, pp.
1290-1291. doi:10.1039/B202177C
[16] Y. Chen, J. F. Gerald, J. S. Williams and S. Bulcock,
“Synthesis of Boron Nitride Nanotubes at Low Tempera-
tures Using Reactive Ball Milling,” Chemical Physics
Letters, Vol. 299, No. 3-4, 1999, pp. 260-264.
doi:10.1016/S0009-2614(98)01252-4
[17] Y. Chen, L. T. Chadderton, J. F. Gerald, J. S. Williams,
“A Solid-State Process for the Formation Boro
Nanotubes,” Applied Physics Letters, Vol. 74
n Nitride
, No. 20,
1999, pp. 2960-2962. doi:10.1063/1.123979
[18] Y. Chen, J. F. Gerald, J. S. Williams and P. Willis, “Me-
chanochemical Synthesis of Boron Nitride Nanotubes,”
Journal of Metastable and Nanocrystalline Materials,
Vol. 2-6, 1999, pp. 173-187.
doi:10.4028/www.scientific.net/JMNM.2-6.173
[19] Y. Chen, M. Conway J. S. Williams and J. Zou, “ Large-
Quantity Production of High-Y
tubes,” Journal of Materials Research, Vol. 17
ield Boron Nitride Nano-
, No. 8,
2002, pp. 1896-1899. doi:10.1557/JMR.2002.0281
[20] S. K. Singhal, A. K. Srivastva, R. P. Pant, S. K. Halder, B.
P. Singh and A. K. Gupta, “Synthesis of Boron Nitride
Nanotubes Employing Mechanothermal Process and Its
Characterization,” Journal of Materials Science, Vol. 43,
No. 15, 2008, pp. 5243-5250.
doi:10.1007/s10853-008-2770-3
[21] J. F. Gerald, Y. Chen and M. J. Conway, “Nanotube Growth
during Annealing of Mechanic
plied Physics A, Vol. 76, No. 1, 2003, pp
ally Milled Boron,” Ap-
.107-110.
doi:10.1007/s00339-002-1441-5
[22] S. Y. Bae, H. W. Seo, J. Park, Y. S. Choi, J. C. Park and
S. Y. Lee, “Boron Nitride Nanotubes Synthesized in
Temperature Range 1000˚C - 1200
The
˚C,” Chemical Physics
Letters, Vol. 374, No. 5-6, 2003, pp. 534-541.
doi:10.1016/S0009-2614(03)00745-0
[23] F. Q. Ji, C. B. Cao, H. Xu and Z. G. Yang, “Mechano-
synthesis of Boron Nitride Nanotubes,” Chines
of Chemical Engineering, Vol. 14, No
e Journal
. 3, 2006, pp. 389-
393. doi:10.1016/S1004-9541(06)60088-8
[24] T. Terao, Y. Bando, M. Mitome, K. Kurashima, C. Y. Zhi,
C. C. Tang and D. Golberg, “Effective Synthesis of Sur-
face-Modified Boron Nitride Nanotubes and Related Nano-
structures and Their Hydrogen Uptake,” Physica E, Vol.
Copyright © 2011 SciRes. WJNSE