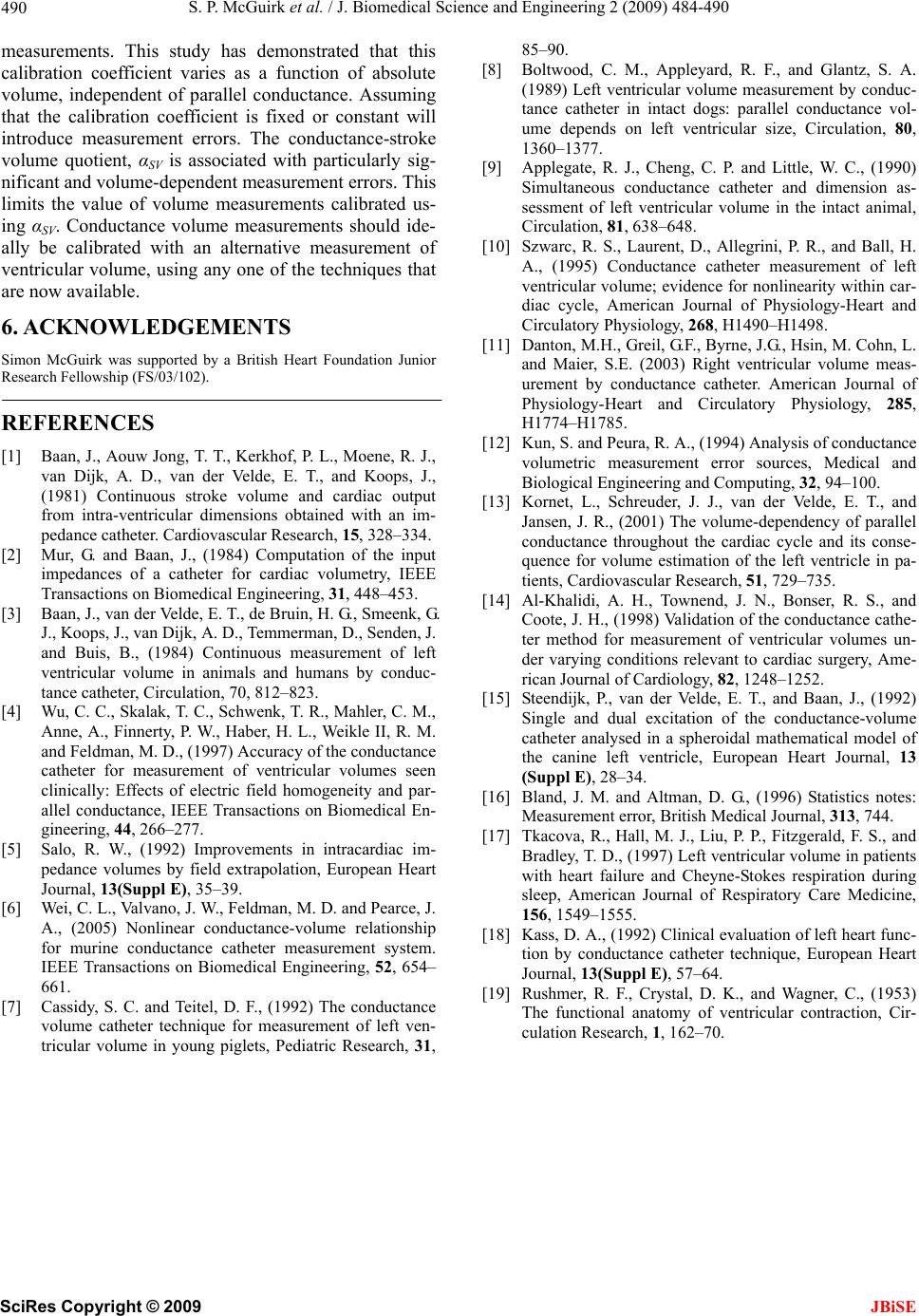
S. P. McGuirk et al. / J. Biomedical Science and Engineering 2 (2009) 484-490
SciRes Copyright © 2009
490
measurements. This study has demonstrated that this
calibration coefficient varies as a function of absolute
volume, independent of parallel conductance. Assuming
that the calibration coefficient is fixed or constant will
introduce measurement errors. The conductance-stroke
volume quotient, αSV is associated with particularly sig-
nificant and volume-dependent measurement errors. This
limits the value of volume measurements calibrated us-
ing αSV. Conductance volume measurements should ide-
ally be calibrated with an alternative measurement of
ventricular volume, using any one of the techniques that
are now available.
JBiSE
6. ACKNOWLEDGEMENTS
Simon McGuirk was supported by a British Heart Foundation Junior
Research Fellowship (FS/03/102).
REFERENCES
[1] Baan, J., Aouw Jong, T. T., Kerkhof, P. L., Moene, R. J.,
van Dijk, A. D., van der Velde, E. T., and Koops, J.,
(1981) Continuous stroke volume and cardiac output
from intra-ventricular dimensions obtained with an im-
pedance catheter. Cardiovascular Research, 15, 328–334.
[2] Mur, G. and Baan, J., (1984) Computation of the input
impedances of a catheter for cardiac volumetry, IEEE
Transactions on Biomedical Engineering, 31, 448–453.
[3] Baan, J., van der Velde, E. T., de Bruin, H. G., Smeenk, G.
J., Koops, J., van Dijk, A. D., Temmerman, D., Senden, J.
and Buis, B., (1984) Continuous measurement of left
ventricular volume in animals and humans by conduc-
tance catheter, Circulation, 70, 812–823.
[4] Wu, C. C., Skalak, T. C., Schwenk, T. R., Mahler, C. M.,
Anne, A., Finnerty, P. W., Haber, H. L., Weikle II, R. M.
and Feldman, M. D., (1997) Accuracy of the conductance
catheter for measurement of ventricular volumes seen
clinically: Effects of electric field homogeneity and par-
allel conductance, IEEE Transactions on Biomedical En-
gineering, 44, 266–277.
[5] Salo, R. W., (1992) Improvements in intracardiac im-
pedance volumes by field extrapolation, European Heart
Journal, 13(Suppl E), 35–39.
[6] Wei, C. L., Valvano, J. W., Feldman, M. D. and Pearce, J.
A., (2005) Nonlinear conductance-volume relationship
for murine conductance catheter measurement system.
IEEE Transactions on Biomedical Engineering, 52, 654–
661.
[7] Cassidy, S. C. and Teitel, D. F., (1992) The conductance
volume catheter technique for measurement of left ven-
tricular volume in young piglets, Pediatric Research, 31,
85–90.
[8] Boltwood, C. M., Appleyard, R. F., and Glantz, S. A.
(1989) Left ventricular volume measurement by conduc-
tance catheter in intact dogs: parallel conductance vol-
ume depends on left ventricular size, Circulation, 80,
1360–1377.
[9] Applegate, R. J., Cheng, C. P. and Little, W. C., (1990)
Simultaneous conductance catheter and dimension as-
sessment of left ventricular volume in the intact animal,
Circulation, 81, 638–648.
[10] Szwarc, R. S., Laurent, D., Allegrini, P. R., and Ball, H.
A., (1995) Conductance catheter measurement of left
ventricular volume; evidence for nonlinearity within car-
diac cycle, American Journal of Physiology-Heart and
Circulatory Physiology, 268, H1490–H1498.
[11] Danton, M.H., Greil, G.F., Byrne, J.G., Hsin, M. Cohn, L.
and Maier, S.E. (2003) Right ventricular volume meas-
urement by conductance catheter. American Journal of
Physiology-Heart and Circulatory Physiology, 285,
H1774–H1785.
[12] Kun, S. and Peura, R. A., (1994) Analysis of conductance
volumetric measurement error sources, Medical and
Biological Engineering and Computing, 32, 94–100.
[13] Kornet, L., Schreuder, J. J., van der Velde, E. T., and
Jansen, J. R., (2001) The volume-dependency of parallel
conductance throughout the cardiac cycle and its conse-
quence for volume estimation of the left ventricle in pa-
tients, Cardiovascular Research, 51, 729–735.
[14] Al-Khalidi, A. H., Townend, J. N., Bonser, R. S., and
Coote, J. H., (1998) Validation of the conductance cathe-
ter method for measurement of ventricular volumes un-
der varying conditions relevant to cardiac surgery, Ame-
rican Journal of Cardiology, 82, 1248–1252.
[15] Steendijk, P., van der Velde, E. T., and Baan, J., (1992)
Single and dual excitation of the conductance-volume
catheter analysed in a spheroidal mathematical model of
the canine left ventricle, European Heart Journal, 13
(Suppl E), 28–34.
[16] Bland, J. M. and Altman, D. G., (1996) Statistics notes:
Measurement error, British Medical Journal, 313, 744.
[17] Tkacova, R., Hall, M. J., Liu, P. P., Fitzgerald, F. S., and
Bradley, T. D., (1997) Left ventricular volume in patients
with heart failure and Cheyne-Stokes respiration during
sleep, American Journal of Respiratory Care Medicine,
156, 1549–1555.
[18] Kass, D. A., (1992) Clinical evaluation of left heart func-
tion by conductance catheter technique, European Heart
Journal, 13(Suppl E), 57–64.
[19] Rushmer, R. F., Crystal, D. K., and Wagner, C., (1953)
The functional anatomy of ventricular contraction, Cir-
culation Research, 1, 162–70.