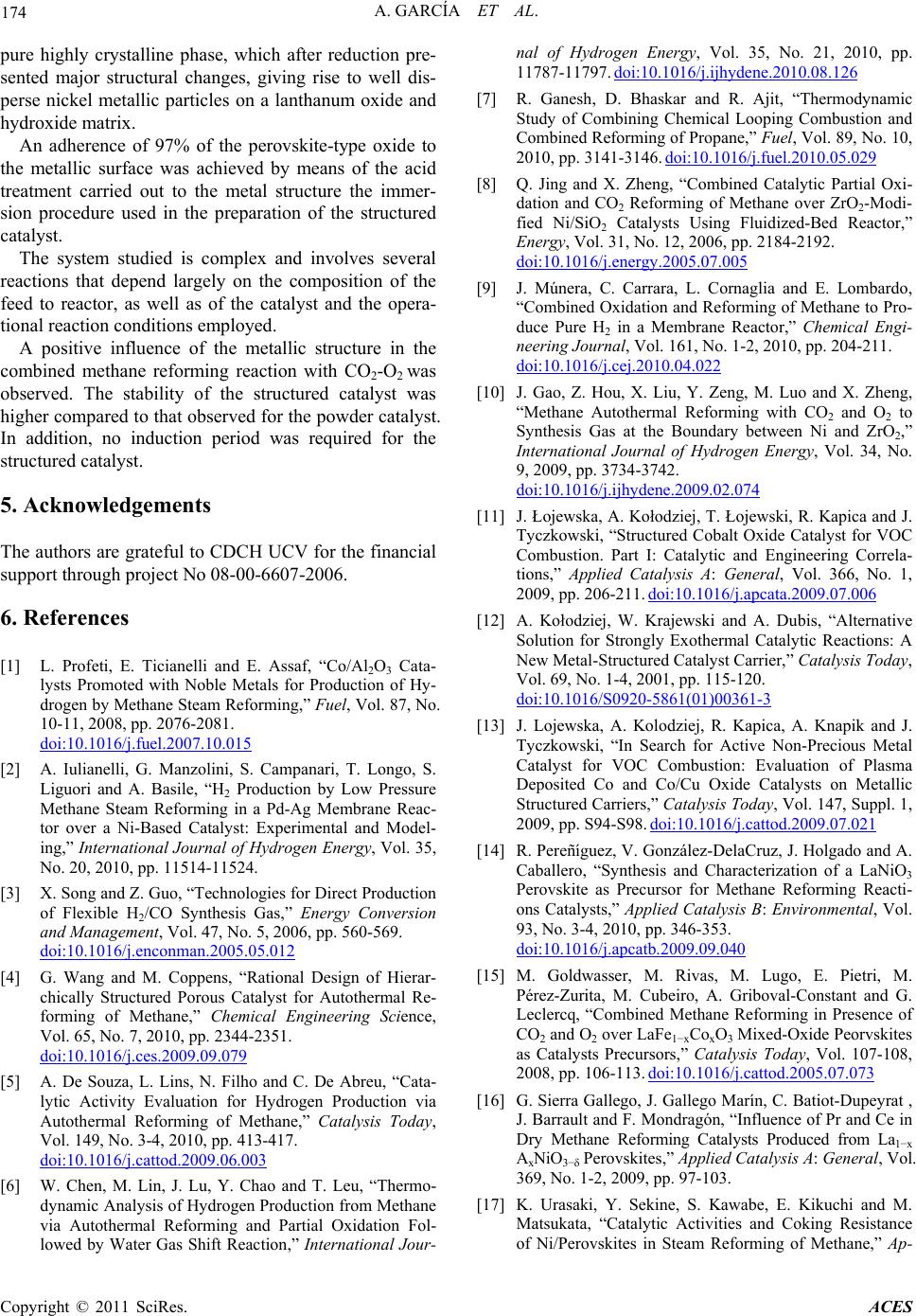
174 A. GARCÍA ET AL.
pure highly crystalline phase, which after reduction pre-
sented major structural changes, giving rise to well dis-
perse nickel metallic particles on a lanthanum oxide and
hydroxide matrix.
An adherence of 97% of the perovskite-type oxide to
the metallic surface was achieved by means of the acid
treatment carried out to the metal structure the immer-
sion procedure used in the preparation of the structured
catalyst.
The system studied is complex and involves several
reactions that depend largely on the composition of the
feed to reactor, as well as of the catalyst and the opera-
tional reaction conditions employed.
A positive influence of the metallic structure in the
combined methane reforming reaction with CO2-O2 was
observed. The stability of the structured catalyst was
higher compared to that observed for the powder catalyst.
In addition, no induction period was required for the
structured catalyst.
5. Acknowledgements
The authors are grateful to CDCH UCV for the financial
support through project No 08-00-6607-2006.
6. References
[1] L. Profeti, E. Ticianelli and E. Assaf, “Co/Al2O3 Cata-
lysts Promoted with Noble Metals for Production of Hy-
drogen by Methane Steam Reforming,” Fuel, Vol. 87, No.
10-11, 2008, pp. 2076-2081.
doi:10.1016/j.fuel.2007.10.015
[2] A. Iulianelli, G. Manzolini, S. Campanari, T. Longo, S.
Liguori and A. Basile, “H2 Production by Low Pressure
Methane Steam Reforming in a Pd-Ag Membrane Reac-
tor over a Ni-Based Catalyst: Experimental and Model-
ing,” International Journal of Hydrogen Energy, Vol. 35,
No. 20, 2010, pp. 11514-11524.
[3] X. Song and Z. Guo, “Technologies for Direct Production
of Flexible H2/CO Synthesis Gas,” Energy Conversion
and Management, Vol. 47, No. 5, 2006, pp. 560-569.
doi:10.1016/j.enconman.2005.05.012
[4] G. Wang and M. Coppens, “Rational Design of Hierar-
chically Structured Porous Catalyst for Autothermal Re-
forming of Methane,” Chemical Engineering Science,
Vol. 65, No. 7, 2010, pp. 2344-2351.
doi:10.1016/j.ces.2009.09.079
[5] A. De Souza, L. Lins, N. Filho and C. De Abreu, “Cata-
lytic Activity Evaluation for Hydrogen Production via
Autothermal Reforming of Methane,” Catalysis Today,
Vol. 149, No. 3-4, 2010, pp. 413-417.
doi:10.1016/j.cattod.2009.06.003
[6] W. Chen, M. Lin, J. Lu, Y. Chao and T. Leu, “Thermo-
dynamic Analysis of Hydrogen Production from Methane
via Autothermal Reforming and Partial Oxidation Fol-
lowed by Water Gas Shift Reaction,” International Jour-
nal of Hydrogen Energy, Vol. 35, No. 21, 2010, pp.
11787-11797. doi:10.1016/j.ijhydene.2010.08.126
[7] R. Ganesh, D. Bhaskar and R. Ajit, “Thermodynamic
Study of Combining Chemical Looping Combustion and
Combined Reforming of Propane,” Fuel, Vol. 89, No. 10,
2010, pp. 3141-3146. doi:10.1016/j.fuel.2010.05.029
[8] Q. Jing and X. Zheng, “Combined Catalytic Partial Oxi-
dation and CO2 Reforming of Methane over ZrO2-Modi-
fied Ni/SiO2 Catalysts Using Fluidized-Bed Reactor,”
Energy, Vol. 31, No. 12, 2006, pp. 2184-2192.
doi:10.1016/j.energy.2005.07.005
[9] J. Múnera, C. Carrara, L. Cornaglia and E. Lombardo,
“Combined Oxidation and Reforming of Methane to Pro-
duce Pure H2 in a Membrane Reactor,” Chemical Engi-
neering Journal, Vol. 161, No. 1-2, 2010, pp. 204-211.
doi:10.1016/j.cej.2010.04.022
[10] J. Gao, Z. Hou, X. Liu, Y. Zeng, M. Luo and X. Zheng,
“Methane Autothermal Reforming with CO2 and O2 to
Synthesis Gas at the Boundary between Ni and ZrO2,”
International Journal of Hydrogen Energy, Vol. 34, No.
9, 2009, pp. 3734-3742.
doi:10.1016/j.ijhydene.2009.02.074
[11] J. Łojewska, A. Kołodziej, T. Łojewski, R. Kapica and J.
Tyczkowski, “Structured Cobalt Oxide Catalyst for VOC
Combustion. Part I: Catalytic and Engineering Correla-
tions,” Applied Catalysis A: General, Vol. 366, No. 1,
2009, pp. 206-211. doi:10.1016/j.apcata.2009.07.006
[12] A. Kołodziej, W. Krajewski and A. Dubis, “Alternative
Solution for Strongly Exothermal Catalytic Reactions: A
New Metal-Structured Catalyst Carrier,” Catalysis Today,
Vol. 69, No. 1-4, 2001, pp. 115-120.
doi:10.1016/S0920-5861(01)00361-3
[13] J. Lojewska, A. Kolodziej, R. Kapica, A. Knapik and J.
Tyczkowski, “In Search for Active Non-Precious Metal
Catalyst for VOC Combustion: Evaluation of Plasma
Deposited Co and Co/Cu Oxide Catalysts on Metallic
Structured Carriers,” Catalysis Today, Vol. 147, Suppl. 1,
2009, pp. S94-S98. doi:10.1016/j.cattod.2009.07.021
[14] R. Pereñíguez, V. González-DelaCruz, J. Holgado and A.
Caballero, “Synthesis and Characterization of a LaNiO3
Perovskite as Precursor for Methane Reforming Reacti-
ons Catalysts,” Applied Catalysis B: Environmental, Vol.
93, No. 3-4, 2010, pp. 346-353.
doi:10.1016/j.apcatb.2009.09.040
[15] M. Goldwasser, M. Rivas, M. Lugo, E. Pietri, M.
Pérez-Zurita, M. Cubeiro, A. Griboval-Constant and G.
Leclercq, “Combined Methane Reforming in Presence of
CO2 and O2 over LaFe1−xCoxO3 Mixed-Oxide Peorvskites
as Catalysts Precursors,” Catalysis Today, Vol. 107-108,
2008, pp. 106-113. doi:10.1016/j.cattod.2005.07.073
[16] G. Sierra Gallego, J. Gallego Marín, C. Batiot-Dupeyrat ,
J. Barrault and F. Mondragón, “Influence of Pr and Ce in
Dry Methane Reforming Catalysts Produced from La1−x
AxNiO3−δ Perovskites,” Applied Cat aly si s A: General, Vol.
369, No. 1-2, 2009, pp. 97-103.
[17] K. Urasaki, Y. Sekine, S. Kawabe, E. Kikuchi and M.
Matsukata, “Catalytic Activities and Coking Resistance
of Ni/Perovskites in Steam Reforming of Methane,” Ap-
Copyright © 2011 SciRes. ACES