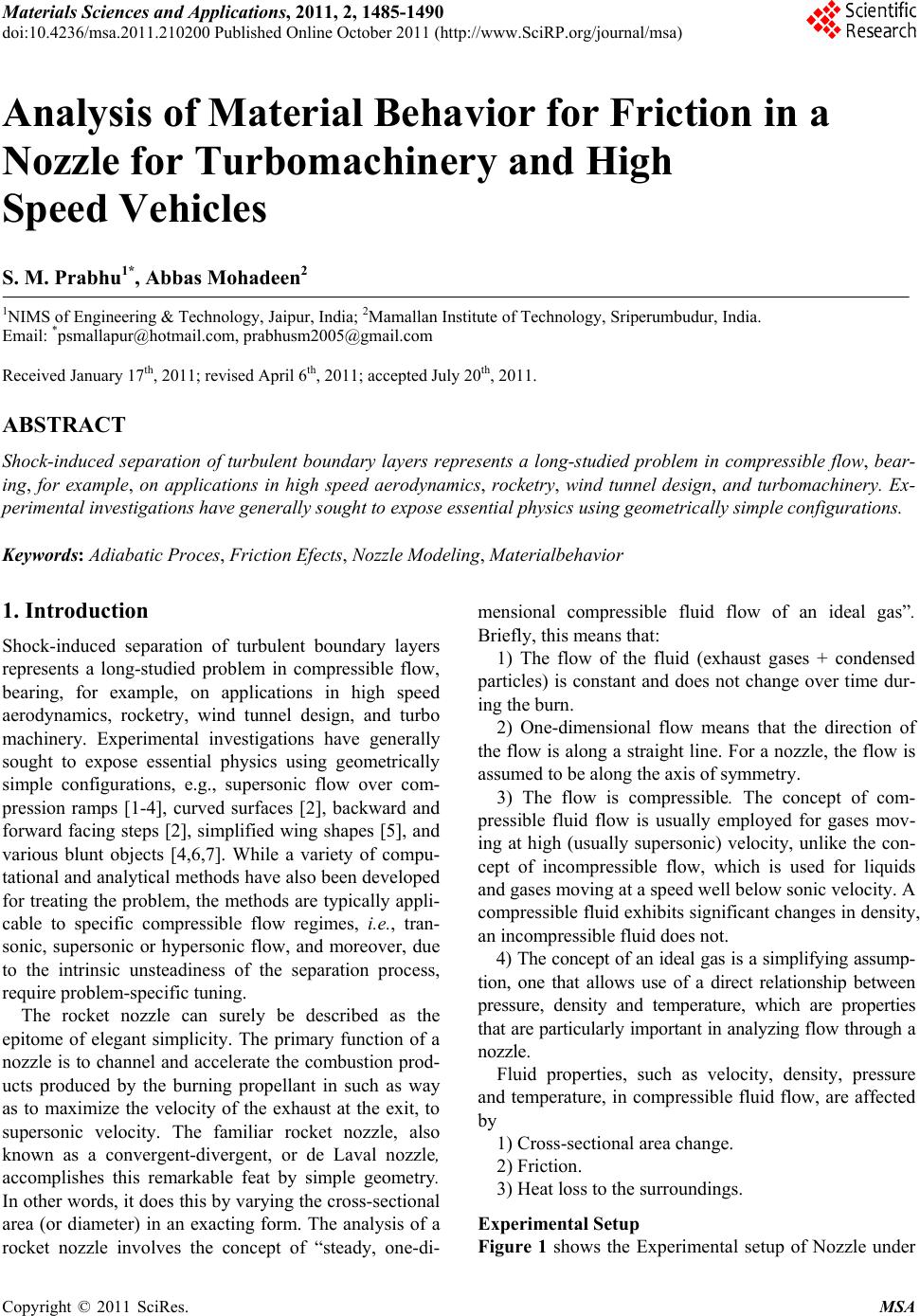
Materials Sciences and Applicatio ns, 2011, 2, 1485-1490
doi:10.4236/msa.2011.210200 Published Online October 2011 (http://www.SciRP.org/journal/msa)
Copyright © 2011 SciRes. MSA
1485
Analysis of Material Behavior for Friction in a
Nozzle for Turbomachinery and High
Speed Vehicles
S. M. Prabhu1*, Abbas Mohadeen2
1NIMS of Engineering & Technology, Jaipur, India; 2Mamallan Institute of Technology, Sriperumbudur, India.
Email: *psmallapur@hotmail.com, prabhusm2005@gmail.com
Received January 17th, 2011; revised April 6th, 2011; accepted July 20th, 2011.
ABSTRACT
Shock-induced separation of turbulent boundary layers represents a long-studied problem in compressible flow, bear-
ing, for example, on applications in high speed aerodynamics, rocketry, wind tunnel design, and turbomachinery. Ex-
perimental investigations have generally sought to expose essential physics using geometrically simple configurations.
Keywords: Adiabatic Proces, Friction Efects, Nozzle Modeling, Materialbehavior
1. Introduction
Shock-induced separation of turbulent boundary layers
represents a long-studied problem in compressible flow,
bearing, for example, on applications in high speed
aerodynamics, rocketry, wind tunnel design, and turbo
machinery. Experimental investigations have generally
sought to expose essential physics using geometrically
simple configurations, e.g., supersonic flow over com-
pression ramps [1-4], curved surfaces [2], backward and
forward facing steps [2], simplified wing shapes [5], and
various blunt objects [4,6,7]. While a variety of compu-
tational and analytical methods have also been developed
for treating the problem, the methods are typically appli-
cable to specific compressible flow regimes, i.e., tran-
sonic, supersonic or hypersonic flow, and moreover, due
to the intrinsic unsteadiness of the separation process,
require problem-specific tuning.
The rocket nozzle can surely be described as the
epitome of elegant simplicity. The primary function of a
nozzle is to channel and accelerate the combustion prod-
ucts produced by the burning propellant in such as way
as to maximize the velocity of the exhaust at the exit, to
supersonic velocity. The familiar rocket nozzle, also
known as a convergent-divergent, or de Laval nozzle,
accomplishes this remarkable feat by simple geometry.
In other words, it does this by varying the cross-sectional
area (or diameter) in an exacting form. The analysis of a
rocket nozzle involves the concept of “steady, one-di-
mensional compressible fluid flow of an ideal gas”.
Briefly, this means that:
1) The flow of the fluid (exhaust gases + condensed
particles) is constant and does not change over time dur-
ing the burn.
2) One-dimensional flow means that the direction of
the flow is along a straight line. For a nozzle, the flow is
assumed to be along the axis of symmetry.
3) The flow is compressible. The concept of com-
pressible fluid flow is usually employed for gases mov-
ing at high (usually supersonic) velocity, unlike the con-
cept of incompressible flow, which is used for liquids
and gases moving at a speed well below sonic velocity. A
compressible fluid exhibits significant changes in density,
an incompressible fluid does not.
4) The concept of an ideal gas is a simplifying assump-
tion, one that allows use of a direct relationship between
pressure, density and temperature, which are properties
that are particularly important in analyzing flow through a
nozzle.
Fluid properties, such as velocity, density, pressure
and temperature, in compressible fluid flow, are affected
by
1) Cross-sectional area change.
2) Friction.
3) Heat loss to the surroundings.
Experimental Setup
Figure 1 shows the Experimental setup of Nozzle under