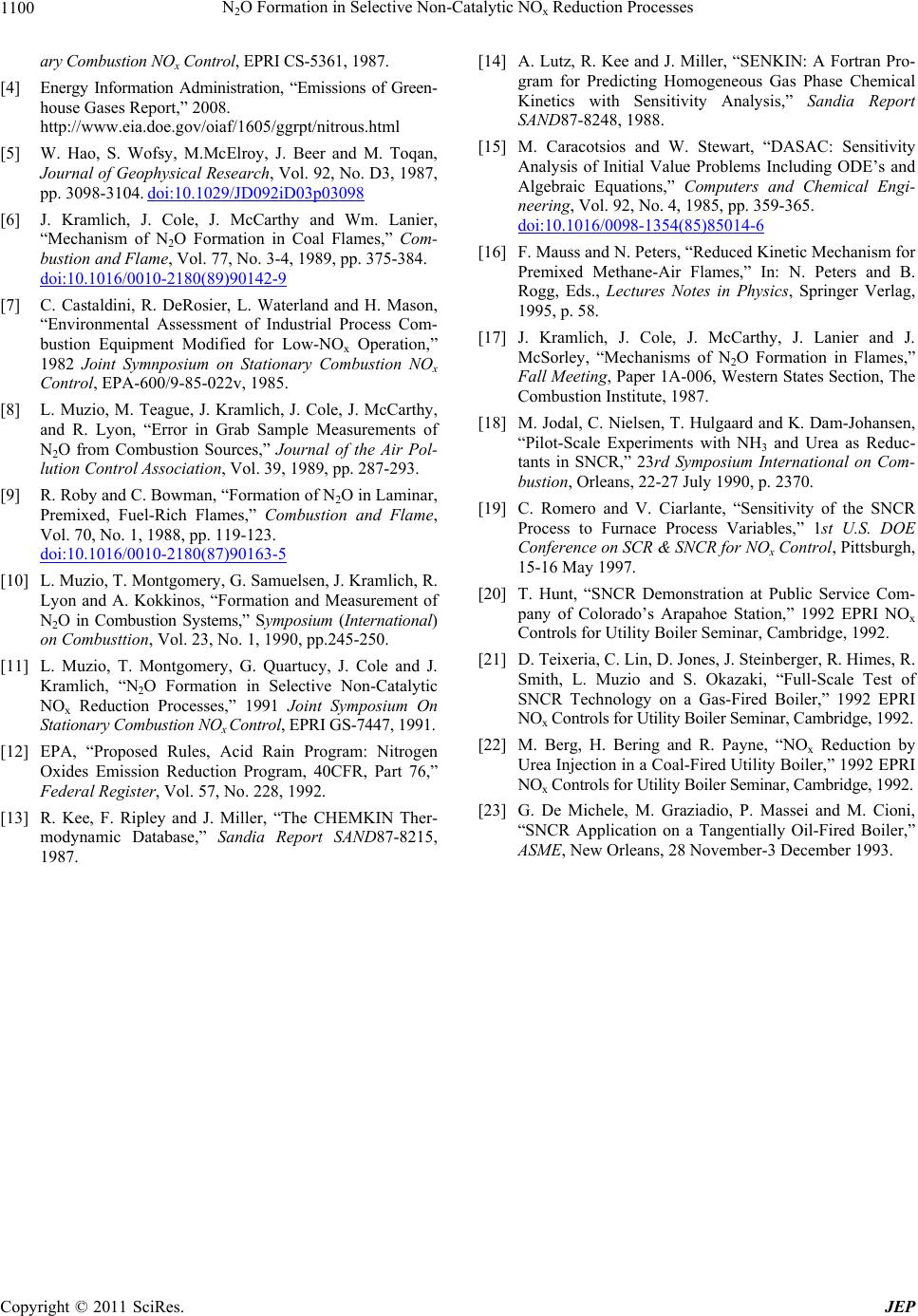
N2O Formation in Selective Non-Catalytic NOx Reduction Processes
Copyright © 2011 SciRes. JEP
1100
ary Combustion NOx Control, EPRI CS-5361, 1987.
[4] Energy Information Administration, “Emissions of Green-
house Gases Report,” 2008.
http://www.eia.doe.gov/oiaf/1605/ggrpt/nitrous.html
[5] W. Hao, S. Wofsy, M.McElroy, J. Beer and M. Toqan,
Journal of Geophysical Research, Vol. 92, No. D3, 1987,
pp. 3098-3104. doi:10.1029/JD092iD03p03098
[6] J. Kramlich, J. Cole, J. McCarthy and Wm. Lanier,
“Mechanism of N2O Formation in Coal Flames,” Com-
bustion and Flame, Vol. 77, No. 3-4, 1989, pp. 375-384.
doi:10.1016/0010-2180(89)90142-9
[7] C. Castaldini, R. DeRosier, L. Waterland and H. Mason,
“Environmental Assessment of Industrial Process Com-
bustion Equipment Modified for Low-NOx Operation,”
1982 Joint Symnposium on Stationary Combustion NOx
Control, EPA-600/9-85-022v, 1985.
[8] L. Muzio, M. Teague, J. Kramlich, J. Cole, J. McCarthy,
and R. Lyon, “Error in Grab Sample Measurements of
N2O from Combustion Sources,” Journal of the Air Pol-
lution Control Association, Vol. 39, 1989, pp. 287-293.
[9] R. Roby and C. Bowman, “Formation of N2O in Laminar,
Premixed, Fuel-Rich Flames,” Combustion and Flame,
Vol. 70, No. 1, 1988, pp. 119-123.
doi:10.1016/0010-2180(87)90163-5
[10] L. Muzio, T. Montgomery, G. Samuelsen, J. Kramlich, R.
Lyon and A. Kokkinos, “Formation and Measurement of
N2O in Combustion Systems,” Symposium (International)
on Combusttion, Vol. 23, No. 1, 1990, pp.245-250.
[11] L. Muzio, T. Montgomery, G. Quartucy, J. Cole and J.
Kramlich, “N2O Formation in Selective Non-Catalytic
NOx Reduction Processes,” 1991 Joint Symposium On
Stationary Combustion NOx Control, EPRI GS-7447, 1991.
[12] EPA, “Proposed Rules, Acid Rain Program: Nitrogen
Oxides Emission Reduction Program, 40CFR, Part 76,”
Federal Register, Vol. 57, No. 228, 1992.
[13] R. Kee, F. Ripley and J. Miller, “The CHEMKIN Ther-
modynamic Database,” Sandia Report SAND87-8215,
1987.
[14] A. Lutz, R. Kee and J. Miller, “SENKIN: A Fortran Pro-
gram for Predicting Homogeneous Gas Phase Chemical
Kinetics with Sensitivity Analysis,” Sandia Report
SAND87-8248, 1988.
[15] M. Caracotsios and W. Stewart, “DASAC: Sensitivity
Analysis of Initial Value Problems Including ODE’s and
Algebraic Equations,” Computers and Chemical Engi-
neering, Vol. 92, No. 4, 1985, pp. 359-365.
doi:10.1016/0098-1354(85)85014-6
[16] F. Mauss and N. Peters, “Reduced Kinetic Mechanism for
Premixed Methane-Air Flames,” In: N. Peters and B.
Rogg, Eds., Lectures Notes in Physics, Springer Verlag,
1995, p. 58.
[17] J. Kramlich, J. Cole, J. McCarthy, J. Lanier and J.
McSorley, “Mechanisms of N2O Formation in Flames,”
Fall Meeting, Paper 1A-006, Western States Section, The
Combustion Institute, 1987.
[18] M. Jodal, C. Nielsen, T. Hulgaard and K. Dam-Johansen,
“Pilot-Scale Experiments with NH3 and Urea as Reduc-
tants in SNCR,” 23rd Symposium International on Com-
bustion, Orleans, 22-27 July 1990, p. 2370.
[19] C. Romero and V. Ciarlante, “Sensitivity of the SNCR
Process to Furnace Process Variables,” 1st U.S. DOE
Conference on SCR & SNCR for NOx Control, Pittsburgh,
15-16 May 1997.
[20] T. Hunt, “SNCR Demonstration at Public Service Com-
pany of Colorado’s Arapahoe Station,” 1992 EPRI NOx
Controls for Utility Boiler Seminar, Cambridge, 1992.
[21] D. Teixeria, C. Lin, D. Jones, J. Steinberger, R. Himes, R.
Smith, L. Muzio and S. Okazaki, “Full-Scale Test of
SNCR Technology on a Gas-Fired Boiler,” 1992 EPRI
NOx Controls for Utility Boiler Seminar, Cambridge, 1992.
[22] M. Berg, H. Bering and R. Payne, “NOx Reduction by
Urea Injection in a Coal-Fired Utility Boiler,” 1992 EPRI
NOx Controls for Utility Boiler Seminar, Cambridge, 1992.
[23] G. De Michele, M. Graziadio, P. Massei and M. Cioni,
“SNCR Application on a Tangentially Oil-Fired Boiler,”
ASME, New Orleans, 28 November-3 December 1993.