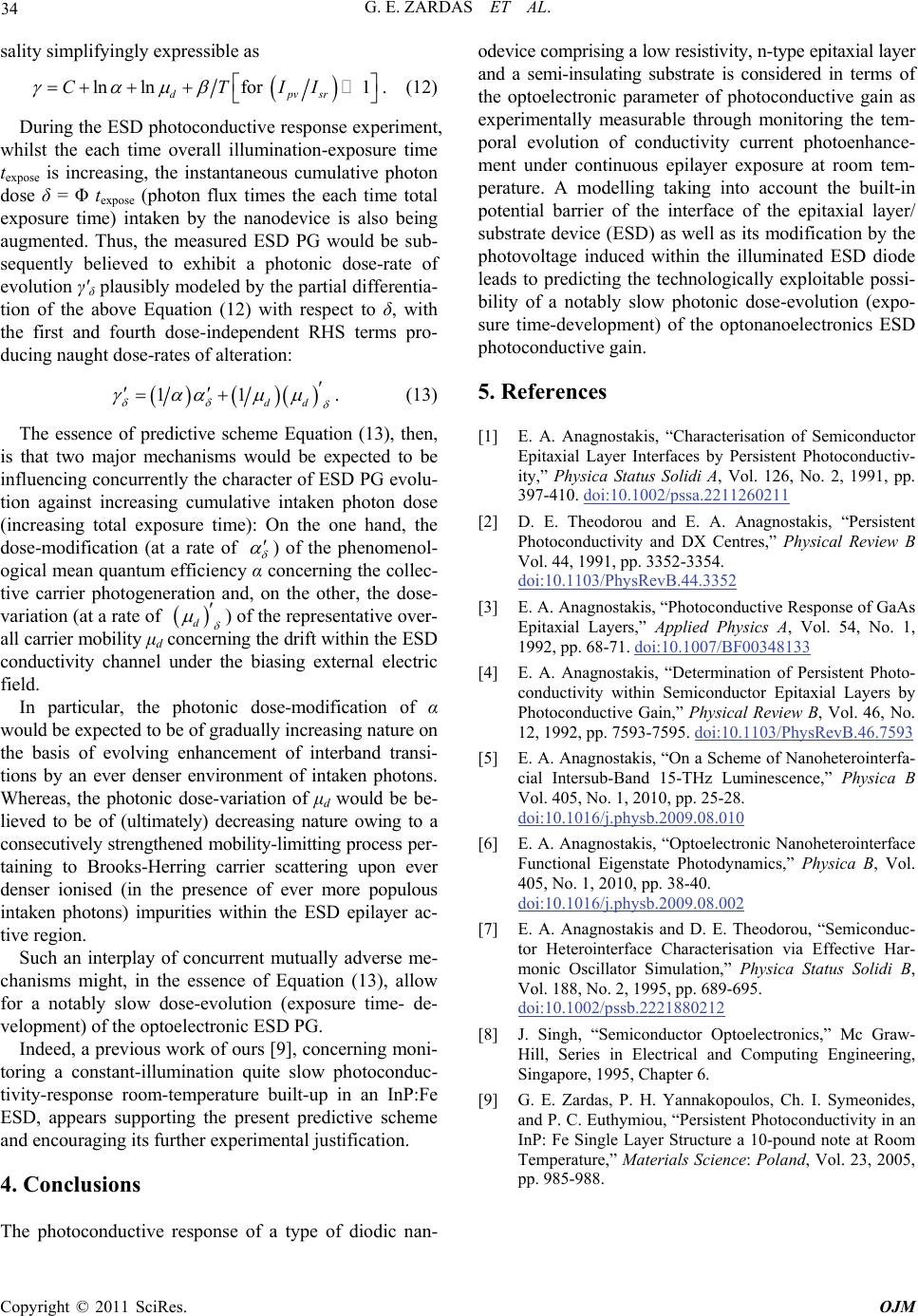
G. E. ZARDAS ET AL.
Copyright © 2011 SciRes. OJM
34
sality simplifyingly expressible as
lnlnfor 1
dpvsr
CTII
. (12)
During the ESD photoconductive response experiment,
whilst the each time overall illumination-exposure time
texpose is increasing, the instantaneous cumulative photon
dose δ = Φ texpose (photon flux times the each time total
exposure time) intaken by the nanodevice is also being
augmented. Thus, the measured ESD PG would be sub-
sequently believed to exhibit a photonic dose-rate of
evolution γ'δ plausibly modeled by the partial differentia-
tion of the above Equation (12) with respect to δ, with
the first and fourth dose-independent RHS terms pro-
ducing naught dose-rates of alteration:
11
dd
. (13)
The essence of predictive scheme Equation (13), then,
is that two major mechanisms would be expected to be
influencing concurrently the character of ESD PG evolu-
tion against increasing cumulative intaken photon dose
(increasing total exposure time): On the one hand, the
dose-modification (at a rate of
) of the phenomenol-
ogical mean quantum efficiency α concerning the collec-
tive carrier photogeneration and, on the other, the dose-
variation (at a rate of
d
) of the representative over-
all carrier mobility μd concerning the drift within the ESD
conductivity channel under the biasing external electric
field.
In particular, the photonic dose-modification of α
would be expected to be of gradually increasing nature on
the basis of evolving enhancement of interband transi-
tions by an ever denser environment of intaken photons.
Whereas, the photonic dose-variation of μd would be be-
lieved to be of (ultimately) decreasing nature owing to a
consecutively strengthened mobility-limitting process per-
taining to Brooks-Herring carrier scattering upon ever
denser ionised (in the presence of ever more populous
intaken photons) impurities within the ESD epilayer ac-
tive region.
Such an interplay of concurrent mutually adverse me-
chanisms might, in the essence of Equation (13), allow
for a notably slow dose-evolution (exposure time- de-
velopment) of the optoelectronic ESD PG.
Indeed, a previous work of ours [9], concerning moni-
toring a constant-illumination quite slow photoconduc-
tivity-response room-temperature built-up in an InP:Fe
ESD, appears supporting the present predictive scheme
and encouraging its further experimental justification.
4. Conclusions
he photoconductive response of a type of diodic nan-
odevice comprising a low resistivity, n-type epitaxial layer
and a semi-insulating substrate is considered in terms of
the optoelectronic parameter of photoconductive gain as
experimentally measurable through monitoring the tem-
poral evolution of conductivity current photoenhance-
ment under continuous epilayer exposure at room tem-
perature. A modelling taking into account the built-in
potential barrier of the interface of the epitaxial layer/
substrate device (ESD) as well as its modification by the
photovoltage induced within the illuminated ESD diode
leads to predicting the technologically exploitable possi-
bility of a notably slow photonic dose-evolution (expo-
sure time-development) of the optonanoelectronics ESD
photoconductive gain.
5. References
[1] E. A. Anagnostakis, “Characterisation of Semiconductor
Epitaxial Layer Interfaces by Persistent Photoconductiv-
ity,” Physica Status Solidi A, Vol. 126, No. 2, 1991, pp.
397-410. doi:10.1002/pssa.2211260211
[2] D. E. Theodorou and E. A. Anagnostakis, “Persistent
Photoconductivity and DX Centres,” Physical Review B
Vol. 44, 1991, pp. 3352-3354.
doi:10.1103/PhysRevB.44.3352
[3] E. A. Anagnostakis, “Photoconductive Response of GaAs
Epitaxial Layers,” Applied Physics A, Vol. 54, No. 1,
1992, pp. 68-71. doi:10.1007/BF00348133
[4] E. A. Anagnostakis, “Determination of Persistent Photo-
conductivity within Semiconductor Epitaxial Layers by
Photoconductive Gain,” Physical Review B, Vol. 46, No.
12, 1992, pp. 7593-7595. doi:10.1103/PhysRevB.46.7593
[5] E. A. Anagnostakis, “On a Scheme of Nanoheterointerfa-
cial Intersub-Band 15-THz Luminescence,” Physica B
Vol. 405, No. 1, 2010, pp. 25-28.
doi:10.1016/j.physb.2009.08.010
[6] E. A. Anagnostakis, “Optoelectronic Nanoheterointerface
Functional Eigenstate Photodynamics,” Physica B, Vol.
405, No. 1, 2010, pp. 38-40.
doi:10.1016/j.physb.2009.08.002
[7] E. A. Anagnostakis and D. E. Theodorou, “Semiconduc-
tor Heterointerface Characterisation via Effective Har-
monic Oscillator Simulation,” Physica Status Solidi B,
Vol. 188, No. 2, 1995, pp. 689-695.
doi:10.1002/pssb.2221880212
[8] J. Singh, “Semiconductor Optoelectronics,” Mc Graw-
Hill, Series in Electrical and Computing Engineering,
Singapore, 1995, Chapter 6.
[9] G. E. Zardas, P. H. Yannakopoulos, Ch. I. Symeonides,
and P. C. Euthymiou, “Persistent Photoconductivity in an
InP: Fe Single Layer Structure a 10-pound note at Room
Temperature,” Materials Science: Poland, Vol. 23, 2005,
pp. 985-988.
T