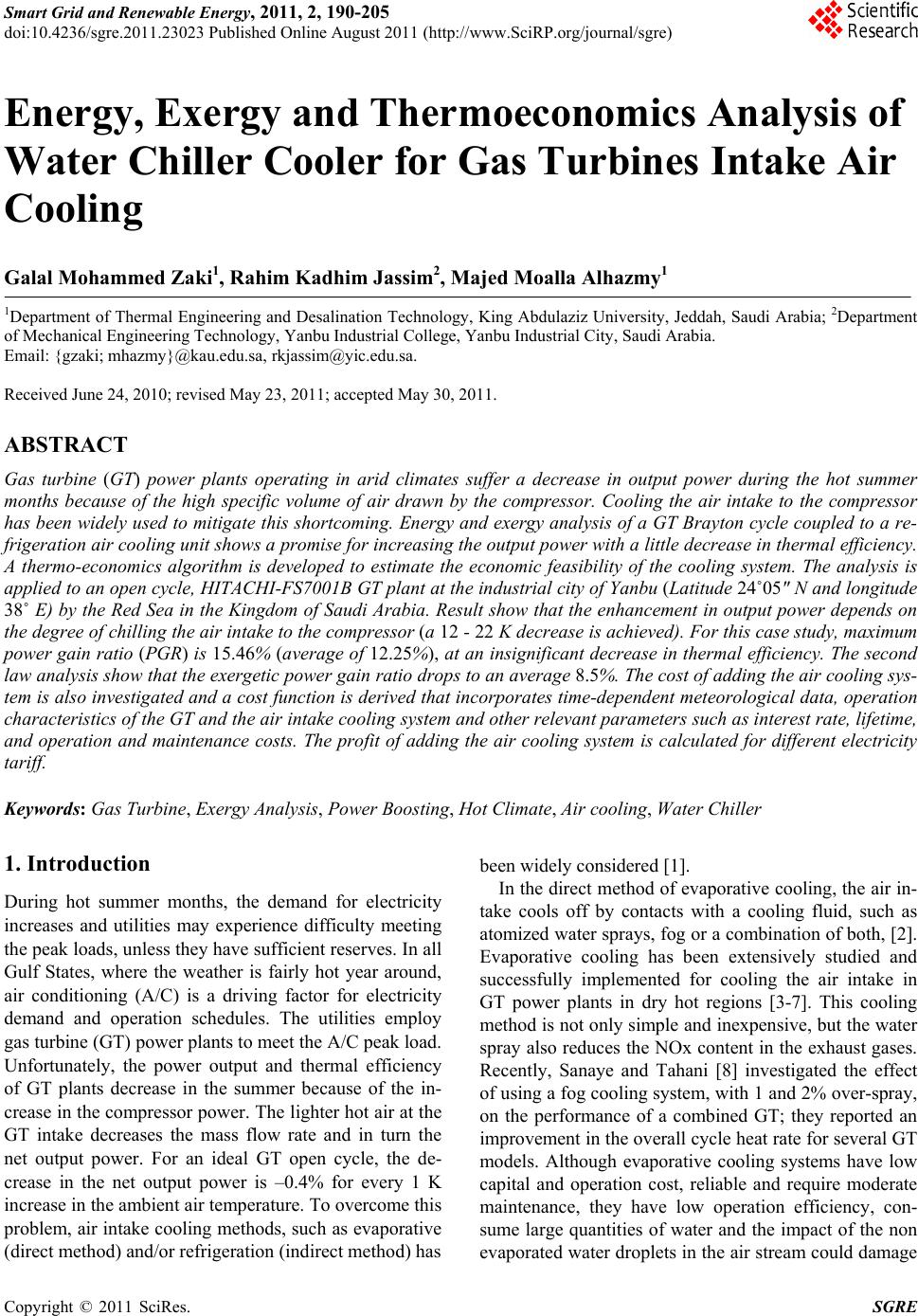 Smart Grid and Renewable Energy, 2011, 2, 190-205 doi:10.4236/sgre.2011.23023 Published Online August 2011 (http://www.SciRP.org/journal/sgre) Copyright © 2011 SciRes. SGRE Energy, Exergy and Thermoeconomics Analysis of Water Chiller Cooler for Gas Turbines Intake Air Cooling Galal Mohammed Zaki1, Rahim Kadhim Jassim2, Majed Moalla Alhazmy1 1Department of Thermal Engineering and Desalination Technology, King Abdulaziz University, Jeddah, Saudi Arabia; 2Department of Mechanical Engineering Technology, Yanbu Industrial College, Yanbu Industrial City, Saudi Arabia. Email: {gzaki; mhazmy}@kau.edu.sa, rkjassim@yic.edu.sa. Received June 24, 2010; revised May 23, 2011; accepted May 30, 2011. ABSTRACT Gas turbine (GT) power plants operating in arid climates suffer a decrease in output power during the hot summer months because of the high specific volume of air drawn by the compressor. Cooling the air intake to the compressor has been widely used to mitigate this shortcoming. Energy and exergy analysis of a GT Brayton cycle coupled to a re- frigeration air cooling unit shows a promise for increasing the output power with a little decrease in thermal efficiency. A thermo-economics algorithm is developed to estimate the economic feasibility of the cooling system. The analysis is applied to an open cycle, HITACHI-FS7001B GT plant at the industrial city of Yanbu (Latitude 24˚05" N and longitude 38˚ E) by the Red Sea in the Kingdom of Saudi Arabia. Result show that the enhancement in output power depends on the degree of chilling the air intake to the compressor (a 12 - 22 K decrease is achieved). For this case study, maximum power gain ratio (PGR) is 15.46% (average of 12.25%), at an insignificant decrease in thermal efficiency. The second law analysis show that the exergetic power gain ratio drops to an average 8.5%. The cost of adding the air cooling sys- tem is also investigated and a cost function is derived that incorporates time-dependent meteorological data, operation characteristics of the GT and the air intake cooling system and other relevant parameters such as interest rate, lifetime, and operation and maintenance costs. The profit of adding the air cooling system is calculated for different electricity tariff. Keywords: Gas Turbine, Exergy Analysis, Power Boosting, Hot Climate, Air cooling, Water Chiller 1. Introduction During hot summer months, the demand for electricity increases and utilities may experience difficulty meeting the peak loads, unless they have sufficient reserves. In all Gulf States, where the weather is fairly hot year around, air conditioning (A/C) is a driving factor for electricity demand and operation schedules. The utilities employ gas turbine (GT) power plants to meet the A/C peak load. Unfortunately, the power output and thermal efficiency of GT plants decrease in the summer because of the in- crease in the compressor power. The lighter hot air at the GT intake decreases the mass flow rate and in turn the net output power. For an ideal GT open cycle, the de- crease in the net output power is –0.4% for every 1 K increase in the ambient air temperature. To overcome this problem, air intake cooling methods, such as evaporative (direct method) and/or refrigeration (indirect method) has been widely considered [1]. In the direct method of evaporative cooling, the air in- take cools off by contacts with a cooling fluid, such as atomized water sprays, fog or a combination of both, [2]. Evaporative cooling has been extensively studied and successfully implemented for cooling the air intake in GT power plants in dry hot regions [3-7]. This cooling method is not only simple and inexpensive, but the water spray also reduces the NOx content in the exhaust gases. Recently, Sanaye and Tahani [8] investigated the effect of using a fog cooling system, with 1 and 2% over-spray, on the performance of a combined GT; they reported an improvement in the overall cycle heat rate for several GT models. Although evaporative cooling systems have low capital and operation cost, reliable and require moderate maintenance, they have low operation efficiency, con- sume large quantities of water and the impact of the non evaporated water droplets in the air stream could damage
 Energy, Exergy and Thermoeconomics Analysis of Water Chiller Cooler for Gas Turbines Intake Air Cooling191 the compressor blades [9]. The water droplets carryover and the resulting damage to the compressor blades, limit the use of evaporative cooling to areas of dry atmosphere. In these areas, the air could not be cooled below the wet bulb temperature (WBT). Chaker, et al. [10-12], Homji- meher, et al. [13] and Gajjar, et al. [14] have presented results of extensive theoretical and experimental studies covering aspects of fogging flow thermodynamics, drop- lets evaporation, atomizing nozzles design and selection of spray systems as well as experimental data on testing systems for gas turbines up to 655 MW in a combined cycle plant. In the indirect mechanical refrigeration cooling ap- proach the constraint of humidity is eliminated and the air temperature can be reduced well below the ambient WBT. The mechanical refrigeration cooling has gained popularity over the evaporative method and in KSA, for example, 32 GT units have been outfitted with mechani- cal air chilling systems. There are two approaches for mechanical air cooling; either using vapor compression (Alhazmy [7] and Elliott [15]) or absorption refrigerator machines (Yang, et al. [16], Ondryas, et al. [17], Pun- wani [18] and Kakarus, et al. [19]). In general, applica- tion of the mechanical air-cooling increases the net power but in the same time reduces the thermal effi- ciency. For example, Alhazmy, et al. [6] showed that for a GT of pressure ratio 8 cooling the intake air from 50˚C to 40˚C increases the power by 3.85% and reduces the thermal efficiency by 1.037%. Stewart and Patrick [20] raised another disadvantage (for extensive air chilling) concerning ice formation either as ice crystals in the chilled air or as solidified layer on air compressors’ en- trance surfaces. Recently, alternative cooling approaches have been investigated. Farzaneh-Gord and Deymi-Dashtebayaz [21] proposed improving refinery gas turbines performance using the cooling capacity of refinerys’ natural-gas pres- sure drop stations. Zaki, et al. [22] suggested a reverse Brayton refrigeration cycle for cooling the air intake; they reported an increase in the output power up to 20%, but a 6% decrease in thermal efficiency. This approach was further extended by Jassim, et al. [23] to include the exergy analysis and show that the second law analysis improvement has dropped to 14.66% due to the compo- nents irreversibilities. Khan, et al. [24] analyzed a system in which the turbine exhaust gases are cooled and fed back to the compressor inlet with water harvested out of the combustion products. Erickson [25,26] suggested using a combination of a waste heat driven absorption air cooling with water injection into the combustion air; the concept is named the “power fogger cycle”. Thermal analyses of GT cooling are abundant in the literature, but few investigations considered the econom- ics of the cooling process. A sound economic evaluation of implementing an air intake GT cooling system is quite involving. Such an evaluation should account for the variations in the ambient conditions (temperature and relative humidity) and the fluctuations in the fuel and electricity prices and interest rates. Therefore, the selec- tion of a cooling technology (evaporative or refrigeration) and the sizing out of the equipment should not be based solely on the results of a thermal analysis but should in- clude estimates of the cash flow. Gareta, et al. [27] has developed a methodology for combined cycle GT that calculated the additional power gain for 12 months and the economic feasibility of the cooling method. From an economical point of view, they provided straight forward information that supported equipment sizing and selec- tion. Chaker, et al. [12] have studied the economical po- tential of using evaporative cooling for GTs in USA, while Hasnain [28] examined the use of ice storage me- thods for GTs’ air cooling in KSA. Yang, et al. [16] pre- sented an analytical method for evaluating a cooling technology of a combined cycle GT that included pa- rameters such as the interest rate, payback period and the efficiency ratio for off-design conditions of both the GT and cooling system. Investigations of evaporative cooling and steam absorption machines showed that inlet fogging is superior in efficiency up to intake temperatures of 15 - 20˚C, though it results in a smaller profit than inlet air chilling [16]. In the present study, the performance of a cooling sys- tem that consists of a chilled water external loop coupled to the GT entrance is investigated. The analysis accounts for the changes in the thermodynamics parameters (ap- plying the first and second law analysis) as well as the economic variables such as profitability, cash flow and interest rate. An objective of the present study is to assess the importance of using a coupled thermo-economics analysis in the selections of the cooling system and op- eration parameters. The developed algorithm is applied to an open cycle, HITACH MS-7001B plant in the hot weather of KSA (Latitude 24˚05" N and longitude 38˚ E) by the result of this case study are presented and dis- cussed. 2. GT-Air Cooling Chiller Energy Analysis Figure 1(a) shows a schematic of a simple open GT “Brayton cycle” coupled to a refrigeration system. The power cycle consists of a compressor, combustion cham- ber and a turbine. It is presented by states 1-2-3-4 on the T-S diagram, Figure 1(b). The cooling system consists of a refrigerant compressor, air cooled condenser, throttle valve and water cooled evaporator. The chilled water from the evaporator passes through a cooling coil mount- ed at the air compressor entrance, Figure 1(a). The re- frigerant cycle is presented on the T-S diagram, Figure 1(c), by states a, b, c and d. A fraction of the power pro- Copyright © 2011 SciRes. SGRE
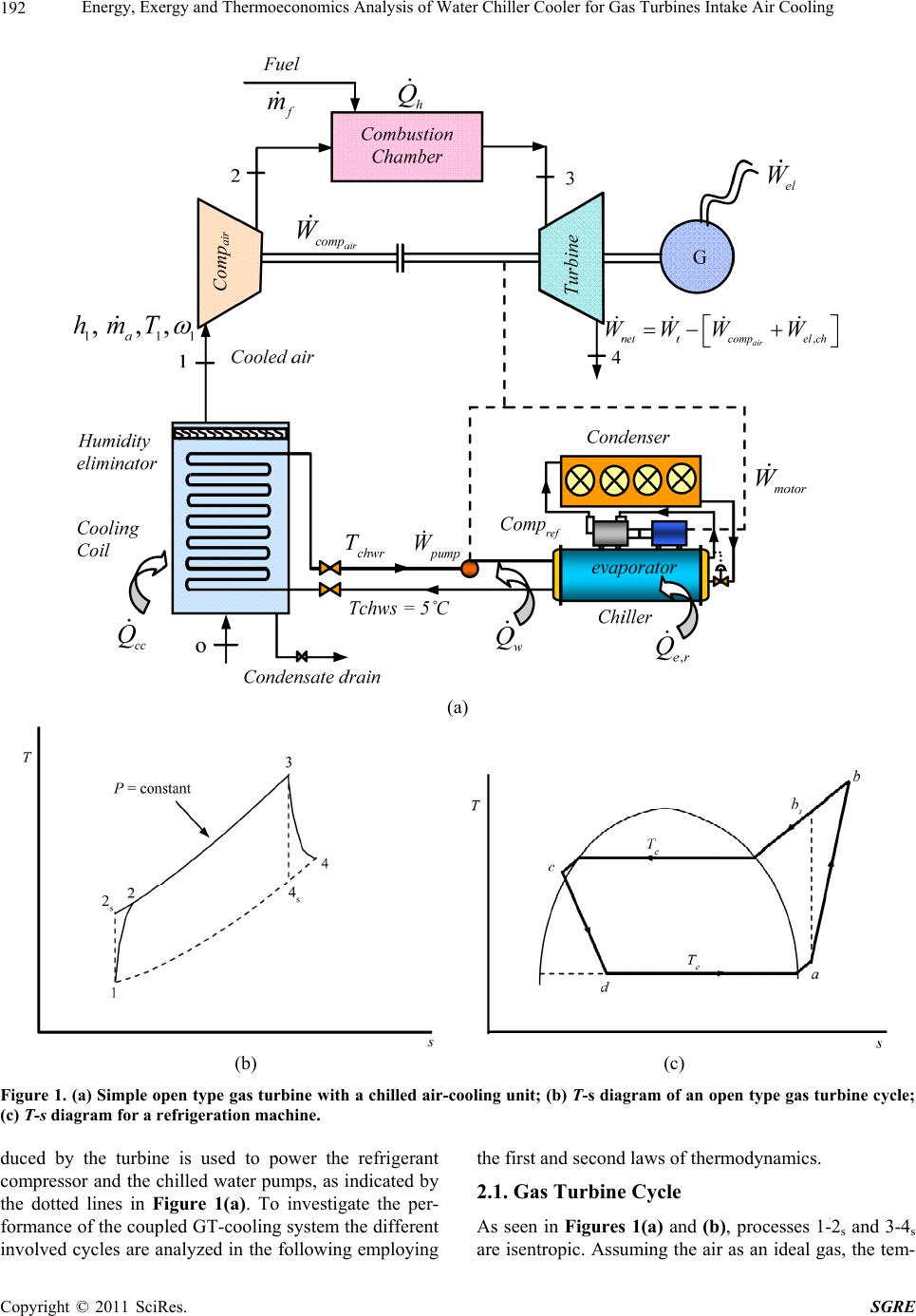 Energy, Exergy and Thermoeconomics Analysis of Water Chiller Cooler for Gas Turbines Intake Air Cooling 192 (a) (b) (c) Figure 1. (a) Simple open type gas turbine with a chilled air-cooling unit; (b) T-s diagram of an open type gas turbine cycle; (c) T-s diagram for a refrigeration machine. duced by the turbine is used to power the refrigerant compressor and the chilled water pumps, as indicated by the dotted lines in Figure 1(a). To investigate the per- formance of the coupled GT-cooling system the different involved cycles are analyzed in the following employing the first and second laws of thermodynamics. 2.1. Gas Turbine Cycle As seen in Figures 1(a) and (b), processes 1-2s and 3-4s are isentropic. Assuming the air as an ideal gas, the tem- C opyright © 2011 SciRes. SGRE
 Energy, Exergy and Thermoeconomics Analysis of Water Chiller Cooler for Gas Turbines Intake Air Cooling193 peratures and pressures are related to the pressure ratio, PR, by: 1 1 23 2 14 1 k k k sk s TT PPR TT P (1) The net power output of a GT with mechanical cooling system as seen in Figures 1(a) is ,nettcompel ch WWW W (2) The first term of the RHS is the power produced by the turbine due to expansion of hot gases; 34ttpgt s Wmc TT (3) In Equation (3), t is the total gases mass flow rate from the combustion chamber; expressed in terms of the fuel air ratio m a mm 1 , and the air humidity ratio at the compressor intake , (kgw/kgdry air) (Figures 1(a)) as; 1 1 tavf a mmmm mf (4) The compression power for humid air between states 1 and 2 is estimated from: 212 1compa pavvv WmcTTmhh (5) where hv2 and hv1 are the enthalpies of saturated water vapor at the compressor exit and inlet states respectively, is the mass of water vapor = v m 1a m . The last term in Equation (2) (,el ch W) is the power consumed by the cooling unit for driving the refrigera- tion machine electric motor, pumps and auxiliaries. The thermal efficiency of a GT coupled to an air cool- ing system is then; ,tcompelch cy h WW W Q (6) Substituting for T4s and from Equations (1) and (4) into Equations (3) yields: t m 13 1 1 11 tapgtk k Wmfc T PR (7) The turbine isentropic efficiency, t , can be estimated using the practical relation recommended by Alhazmy and Najjar [6]: 1 10.03180 t PR actual compressor power becomes; (8) Relating the compressor isentropic efficiency to the changes in temperature of the dry air and assuming that the compression of water vapor changes the enthalpy; the 1 1 Tk k 12 1 c 1 η air comp a pavv WmcPR hh (9) The compression efficiency, c , can be evaluated us- ing the following empirical relat, Alhazmy and Najjar [6]; ion 1 10.04 150 c PR (11) The heat balance in the combustion chamber ( 1( 2 (12) Introducing the fuel air ratio Figure a)) gives the heat rate supplied to the gas power cycle as: 323 hf comb afpg apa vvv Qm NCV mmcTmcTmh h a mm and substi- tu quation (12ting for T2 in terms of T1 into E) yields: ha1 QmT k1 k 31 pg pav3 v2 1c1 Tω PR 1 1fcc1h h TηT (13) A simple expression for is selected here, Alha et zmy, al. [7] as: 3213 3 298 298 298 pgpav v comb pg 2 cTh h fNCV c T (14) In Equation (14), hv2 and hv3 are the enthalpies of water va i + 1.8723 Tj j refers to states 2 or 3 (15) ci is the cT por at the combuston chamber inlet and exit states respectively and can be calculated from Equation (15), Dossat [29]. hv,j= 2501.3 The four terms of the gas turbine net power and effi- ency in Equation (6) (, tcomp WW ,,el ch W and h Q ) depend on the air temperature aneidity the com- pressor inlet whose values are affected by the type and performance of the cooling system. The chillers’ electric power, ,el ch W , is calculated in the following account. 2.2. Refrigeration Cooling System Analysis d relativ humat The chilled water from the refrigeration machine heat transport fluid to cool the intake air, Figure 1(a). The chiller’s total electrical power can be expressed as the sum of the electric motor power (motor W ), the pumps ( W ) and auxiliary power for fans and control units, (A W ) as: ,el chmotorPA WWWW (16) Copyright © 2011 SciRes. SGRE
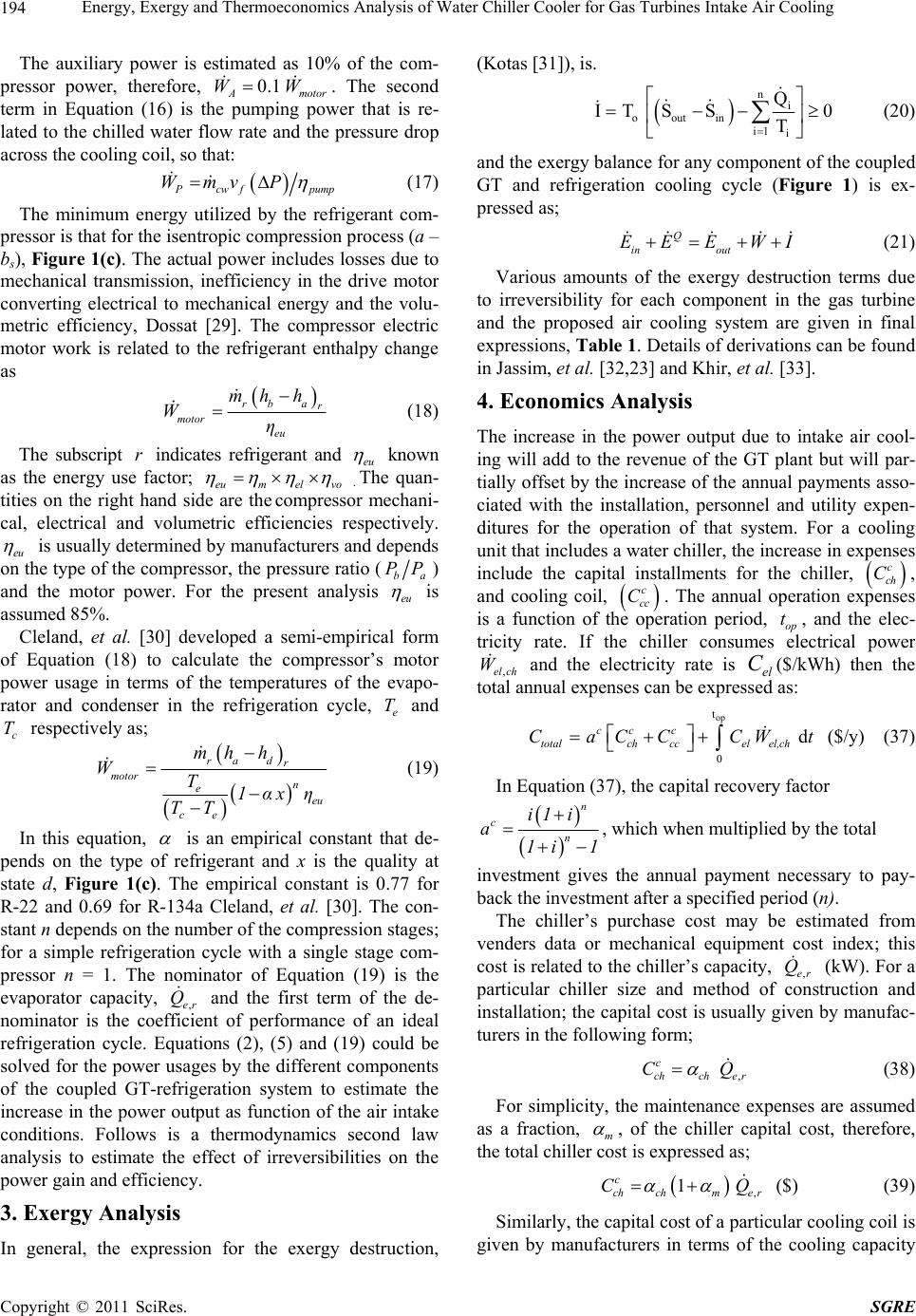 Energy, Exergy and Thermoeconomics Analysis of Water Chiller Cooler for Gas Turbines Intake Air Cooling 194 The auxiliary power is estimated as 10% press of the com- or power, therefore, 0.1 A motor WW. The second term in Equation (16) is the pumping power that is re- lated to the chilled water flow rate and the pressure drop across the cooling coil, so that: cw f Wmv P (17) pump The minimum energy utilized by the ref press rigerant com- or is that for the isentropic compression process (a – bs), Figure 1(c) . The actual power includes losses due to mechanical transmission, inefficiency in the drive motor converting electrical to mechanical energy and the volu- metric efficiency, Dossat [29]. The compressor electric motor work is related to the refrigerant enthalpy change as rb a r motor eu mh h Wη (18) The subscript indicates refrigerant an r d eu known as the fact energy useor; eum el vo . tities on the right hand side are the compressor mechani- cal, electrical and volumetric effiespectively. eu The quan- ciencies r is usually determined by manufacturers and depends on the type of the compressor, the pressure ratio (ba PP) and the motor power. For the present analysis eu is assumed 85%. Cleland, et al. [30] developed a semi-empiricalrm of Equation (1 fo 8) to calculate the compressor’s motor power usage in terms of the temperatures of the evapo- rator and condenser in the refrigeration cycle, e T and c T respectively as; rad r motor mh h W n e eu ce T1αxη TT (19) In this equation, is an empirical constant that de- pen on for the exergy destruction, ds on the type of refrigerant and x is the quality at state d, Figure 1(c). The empirical constant is 0.77 for R-22 and 0.69 for R-134a Cleland, et al. [30]. The con- stant n depends on the number of the compression stages; for a simple refrigeration cycle with a single stage com- pressor n = 1. The nominator of Equation (19) is the evaporator capacity, ,er Q and the first term of the de- nominator is the coefficient of performance of an ideal refrigeration cycle. Equations (2), (5) and (19) could be solved for the power usages by the different components of the coupled GT-refrigeration system to estimate the increase in the power output as function of the air intake conditions. Follows is a thermodynamics second law analysis to estimate the effect of irreversibilities on the power gain and efficiency. 3. Exergy Analysis In general, the expressi (Kotas [31]), is. n i ooutin i1 i Q IT SS0 T (20) and the exergy balance for any component of the coupled GT and refrigeration cooling cycle (Figure 1) is ex- pressed as; Q in out EE E WI (21) Various amounts of the exergy destru to tput due to intake air cool- ction terms due irreversibility for each component in the gas turbine and the proposed air cooling system are given in final expressions, Table 1. Details of derivations can be found in Jassim, et al. [32,23] and Khir, et al. [33]. 4. Economics Analysis The increase in the power ou ing will add to the revenue of the GT plant but will par- tially offset by the increase of the annual payments asso- ciated with the installation, personnel and utility expen- ditures for the operation of that system. For a cooling unit that includes a water chiller, the increase in expenses include the capital installments for the chiller, c ch C, and cooling coil, c cc C. The annual operation exp is a function of thration period, op t, and the elec- tricity rate. If the chiller consumes electrical power ,el ch W and the electricity rate is el C($/kWh) then the nnual expenses can be expresas: op t enses e ope total ased 0 d cc c totalchccel el,ch CaCC CW t($/y) (37) In Equation (37), the capital recovery factor n i1 i c n a 1i 1 , which when multiplied by the total investment gives the annual payment necessary to pay- ed from ve Q (38) For simplicity, the maintenance expe as back the investment after a specified period (n). The chiller’s purchase cost may be estimat nders data or mechanical equipment cost index; this cost is related to the chiller’s capacity, ,er Q (kW). For a particular chiller size and method of ruction and installation; the capital cost is usually given by manufac- turers in the following form; c C const , ch che r nses are assumed a fraction, m , of the chiller capital cost, therefore, the total chillerst is expressed as; co 1 c CQ ,ch chme r ($) (39) Similarly, the capital cost of a particu gi lar cooling coil is ven by manufacturers in terms of the cooling capacity C opyright © 2011 SciRes. SGRE
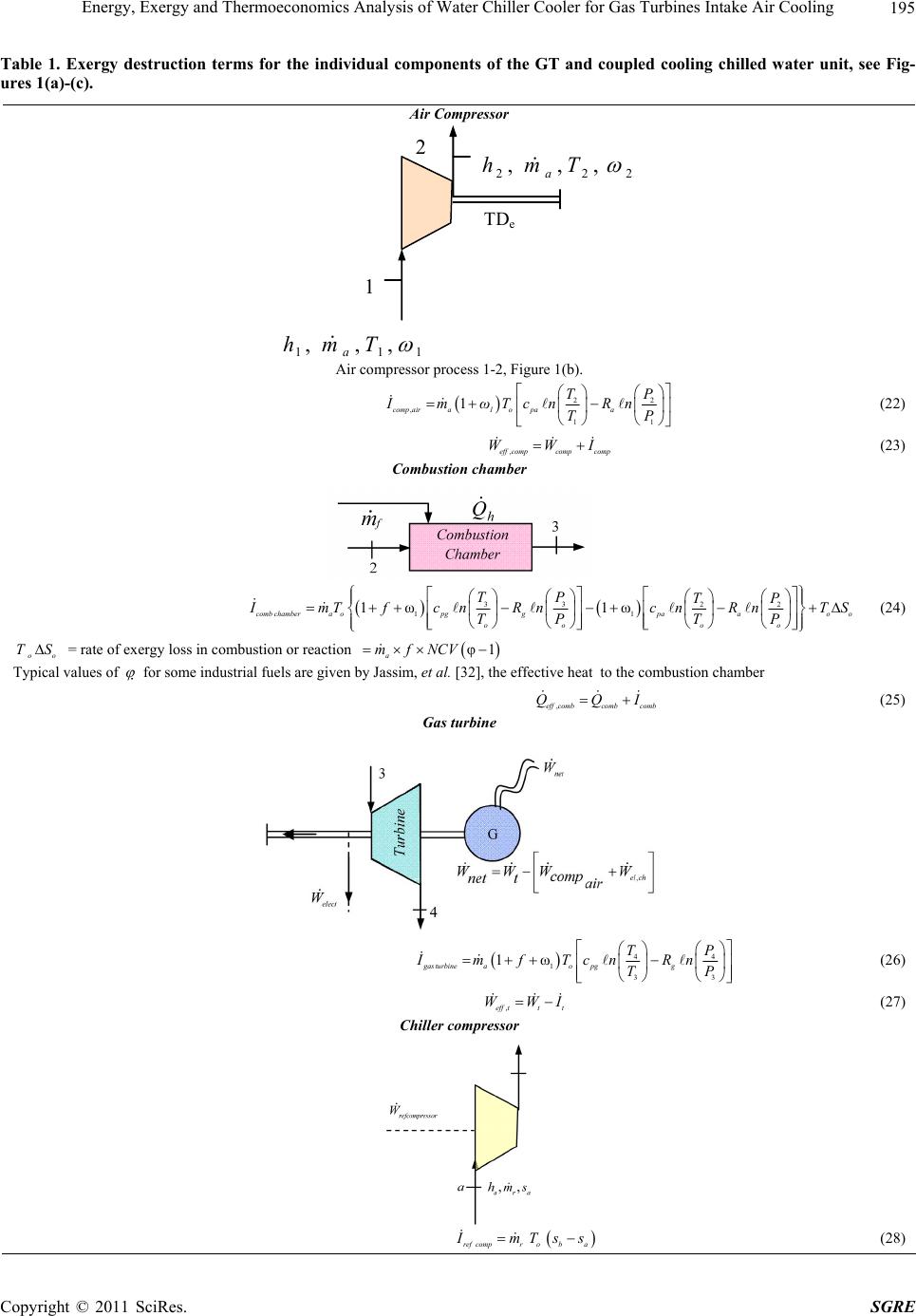 Energy, Exergy and Thermoeconomics Analysis of Water Chiller Cooler for Gas Turbines Intake Air Cooling Copyright © 2011 SciRes. SGRE 195 nts of the GT and coupled cooling chilled water unit, see Fig- Air Compressor Table 1. Exergy destruction terms for the individual compone ures 1(a)-(c). TD e 1 111 ,,, Tmh a 2 222 ,,, Tmh a Air compressor process 1-2, Figure 1(b). 2 1T ImωTc n2 , 11 comp aira1opaa P R n TP (22) (23) Combustion chamber ,eff compcompcomp WWI 33 22 11 1ω1ω combchamberaopggpaaoo oo oo TP TP mTfcnRncnRn T TP TP S (24) = rate of exergy loss in combustion or reaction oo TS φ1 a mfNCV Typical values of for some industrial fuels are given by Jassim, et al. [32], the effective heat to the combustion chamber (25) Gas turbine ,eff combcombcomb QQI 44 1 3 1ω gas turbineaopgg TP ImfTcnRn T3 P (26) I ,eff ttt WW (27) Chiller compressor ref comprbao mT ss (28)
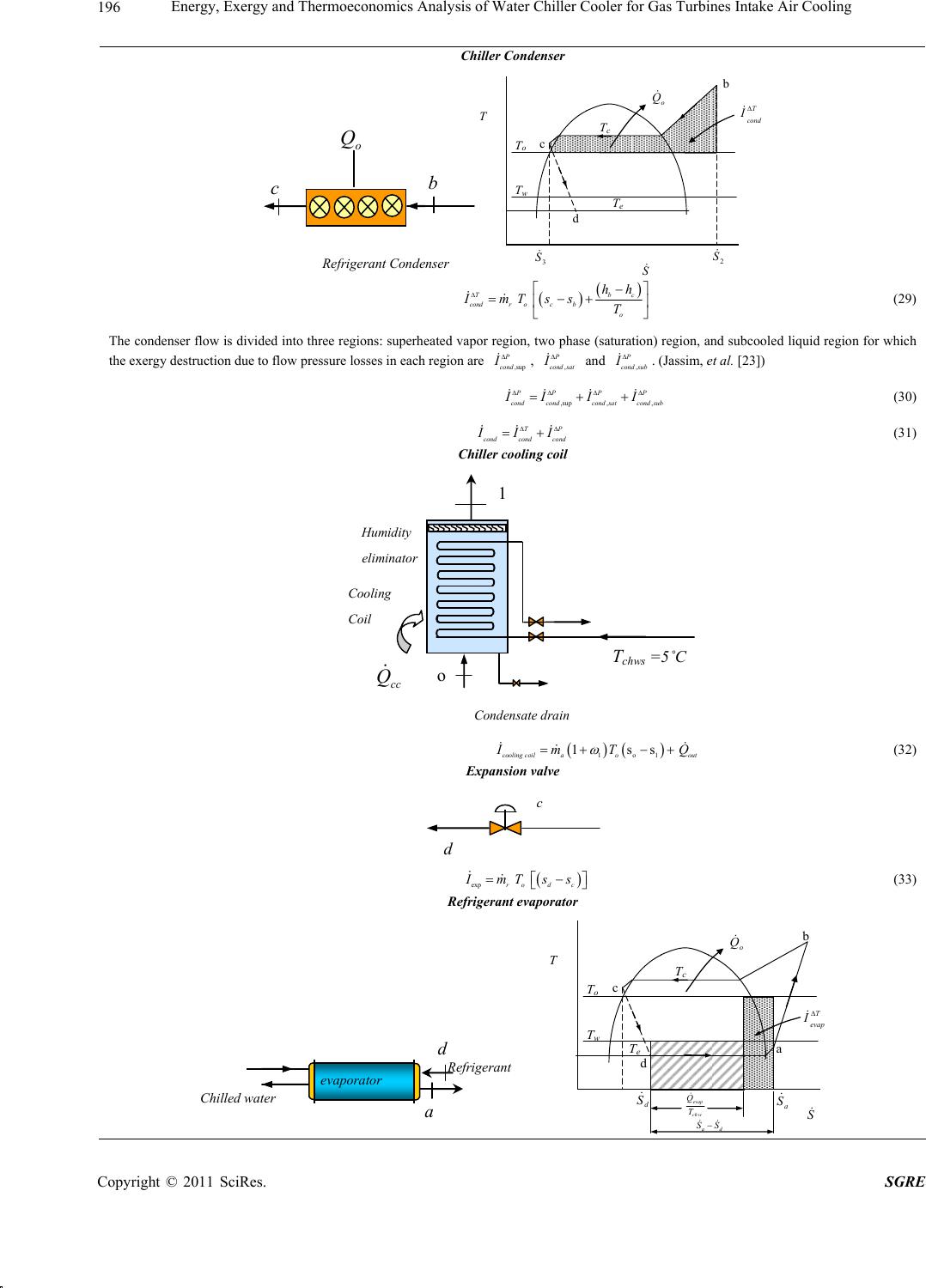 Energy, Exergy and Thermoeconomics Analysis of Water Chiller Cooler for Gas Turbines Intake Air Cooling 196 Chiller Condenser efrigerant Condense b c o Q S T o b 3 S 2 S T cond I T T w T c c d T e o Q Tb condr ocb o hh ImTss T c (29) The condenser flow is divided into three regions: superheated vapor region, two phase (saturation) region, and subcooled liquid region for which (30) the exergy destruction due to flow pressure losses in each region are ,sup P cond I , , P cond sat I and , P cond sub I . (Jassim, et al. [23]) P ,sup ,, PPP condcondcond satcond sub III I TP condcond cond II Chiller cooling coil (31) Humidity eliminator Cooling Coil o cc Q Condensate drain T chws =5˚C 1 1o1 1ss cooling coilaoout mT Q (32) Expansion valve c d exp rod c ImTss (33) Refrigerant evaporator Chilled water evaporator efrigeran d a S T o b d S a S T evap I T T w c c d T e o Q wch evap T Q da SS C opyright © 2011 SciRes. SGRE
 Energy, Exergy and Thermoeconomics Analysis of Water Chiller Cooler for Gas Turbines Intake Air Cooling Copyright © 2011 SciRes. SGRE 197 Ta evapr oad sw hh ImTss T d (34) The refrigerant flow in the evaporator is divided into two regimes saturation (two phase) and superheated regions. The two phase (saturation) region, and superheated vapor region for which the exergy destruction due to flow pressure losses in each region are see Khir, et al. [33]. The exergy destruction rate is the sum of the thermal and pressure loss terms for both regimes (Equations (35) and (36)) as, , P evapsat I , ,sup P evap I TP evapevapevap II (35) sup (36) ,, PP P evapevapsatevap II I that is directly proportional to the total heat transfer sur- face area ( cooling coil to enter the air compressor intake at and , Figure 1(a). Both and dpend the illed water su Wh va the rocmay r 1 T on ess , m2) Kotas [31]) as, ($) (40) cc 1eω ch rate, be t trated i ing c 1 T1 ω po pply temperature (Tchws) and mass flow en the outer surface temperature of the m c cccccc CA cw m . ea n oil gi cooling coil falls below the dew point (corresponding to the partial pressure of the water r)water vapor condensates and leaves the air stream. This p ted as a cooling-dehumidification process as illus- Figure 3. Steady state heat balance of the cool- ves; In Equation (40), cc and m depend on the type of e cooling coil and material. For the present study and cal Saudi market, cc th lo = 30000 and m= 0.582 are recommended (Yorltation [3bstituting Equations (39) and (40) into Equation (37), assuming for mplicity that the chiller power is an average constant nn he k Co consu4]). Su si value and constant electricity rate over the operation pe- riod, the aual total expenses for the cooling system become; 1ccaow wcw Qmhhmhm w chwr T 1 c totalchme rccccopelelch CaQA tCW m ,, ( $/y) (41) In Equation (41) the at transfer area cc ris the chilledr water extractiofrom th ,chws T eff cc c mass flow e air, (45) ate and w m whe is th e, cw m e rate of water n 1wao mm . The second term in Equation (45) is d can be negl usually a sm ecte ng all term when cmp the first a d, McQuiston, [35] In Equation (45) the entalpy and temperature of the o et al. h ared to . n air leavithe cooling coil (h1 and T1) may be calculated from; 1oos hhCFhh (46) 1oos TTCFT (47) The contact factor CF is defined as the ratio between the actual air temperature drop to the maximum, at which the air theatrically leaves at the coil surface temperature Ts = Tchws and 100% relative humidity. Substituting for h1 from Equation (46) into Equation (45) and use Equation (42) gives; is the pa- rameter used to evaluate the cost of the cooling coil. En- ergy balance on both the cooling coil and the refrigerant evaporator, taking into account the effectiveness factors for the evaporator, ,eff er , and the cooling coil, ,eff cc , gives T ,, , ereff er cc cc meffcc m Q Q AUTFUTF (4 where, U is the overall heat tran ch G coil d chilled water fluids) is; 2) sfer coefficient for illed water-air tube bank heat exchanger. areta, et al. [27] suggested a moderate value of 64 W/m2 K and 0.98 for the correction factor F. Figure 2, illustrates the temperature variations in the combined refrigerant, water chiller and air cooling sys- tem. the mean temperature difference for the cooling (air an 1 1 1 ochwr chws nT TT T Equations (40) and (42) give the cooling coil cost as, o chwrchws m TT TT T (43) m cQ C cc (44) cc cc m UT F where, cc Q is the thermal capacity of the cooling coil. The atmospheric air enters at To and o and leaves the , Q ,, aochwso er r eff mCFh h w eff e h cc 4) s o e otal annual cotermv , as, n t er Q ( aporator ca- 8 Equatio (41) through (48) give the chiller and c ing coil annual expenses in terms of the air mass flow rate and propertis. The total annual cost function is de- rived from Equation (41) as follows. 4.1. Annual Cost Function o l- Combining Equations (41) and (42), substituting for the cooling coil surface area, pump and auxiliary power gives thest in s of the e pacity
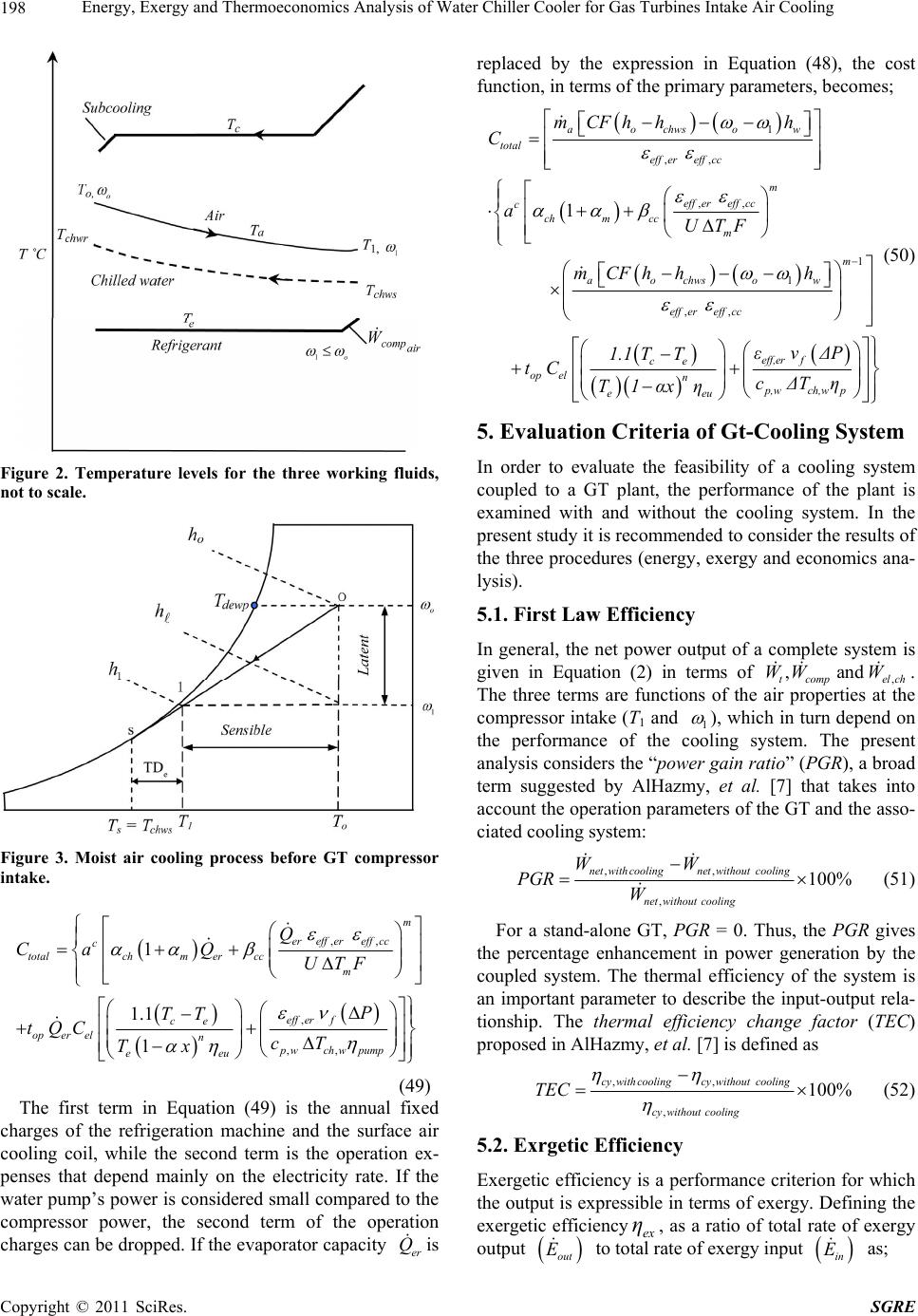 Energy, Exergy and Thermoeconomics Analysis of Water Chiller Cooler for Gas Turbines Intake Air Cooling 198 Figure 2. Temperature levels for the three working fluids, not to scale. Figure 3. Moist air cooling process before GT compressor intake. ,, , ,, 1ereff ereff cc c totalchm ercc Ca QUTF 1.1 1 m m eff erf ce oper eln p wch wpump eeu Q P TT tQC cT Tx (49) The first term in Equation (49) is the annual fixed charges of the refrigeration machine and the surface air cooling coil, while the second term is the operation ex- penses that depend mainly on the electricity rate. If the water pump’s power is considered small compared to the compressor power, the second term of the operation charges can be dropped. If the evaporator capacity is replaced by the expression in Equation (48), the cost function, in terms of the primary parameters, becomes; er Q 1 ,, ,, 1 1 ,, 1 aochwsow total eff ereffcc m eff ereff cc c chm cc m m aochwsow eff ereffcc eff ,er ce mCFh hh C aUTF mCFh hh εν 1.1 TT tC op el n eeu T1αxη fΔP p,w ch,w p cΔTη (50) 5. Evaluation Criteria of Gt-Cooling System In order to evaluate the feasibility of a cooling system coupled to a GT plant, the performance of the plant is examined with and without the cooling system. In t present study it is recommended to consider the results of the three procedures (energy, exergy and economics ana- lysis). 5.1. First Law Efficiency he In general, the net power output of a complete system is given in Equation (2) in terms of , ,and tcomp elch WW W . The three terms are functions of the air properties at the compressor intake (T1 and 1 ), which in turn depend on the performance of the cooling system. The present analysis considers the “power gain ratio” (PGR), a broad term suggested by AlHazmy, et al. [7] that takes into account the operation parameters of the GT and the asso- ciated cooling system: ,, , 100% net withcoolingnetwithout cooling netwithout cooling WW PGR W (51) For a stand-alone GT, PGR = 0. Thus, the PGR gives the percentage enhancement in power generation by the coupled system. The thermal efficiency of the syste an important parameter to describe the input-output tionship. The thermal efficiency change factor (TEC) proposed in AlHazmy, et al. [7] is defined as m is rela- ,, , 100% cy withcoolingcy withoutcooling cy without cooling TEC (52) 5.2. Exrgetic Efficiency Exergetic efficiency is a performance criterion for which the output is expressible in terms of exergy. Defining the exergetic efficiencyex η, as a ratio of total rate of exergy output out E to total rate of exergy input in E as; C opyright © 2011 SciRes. SGRE
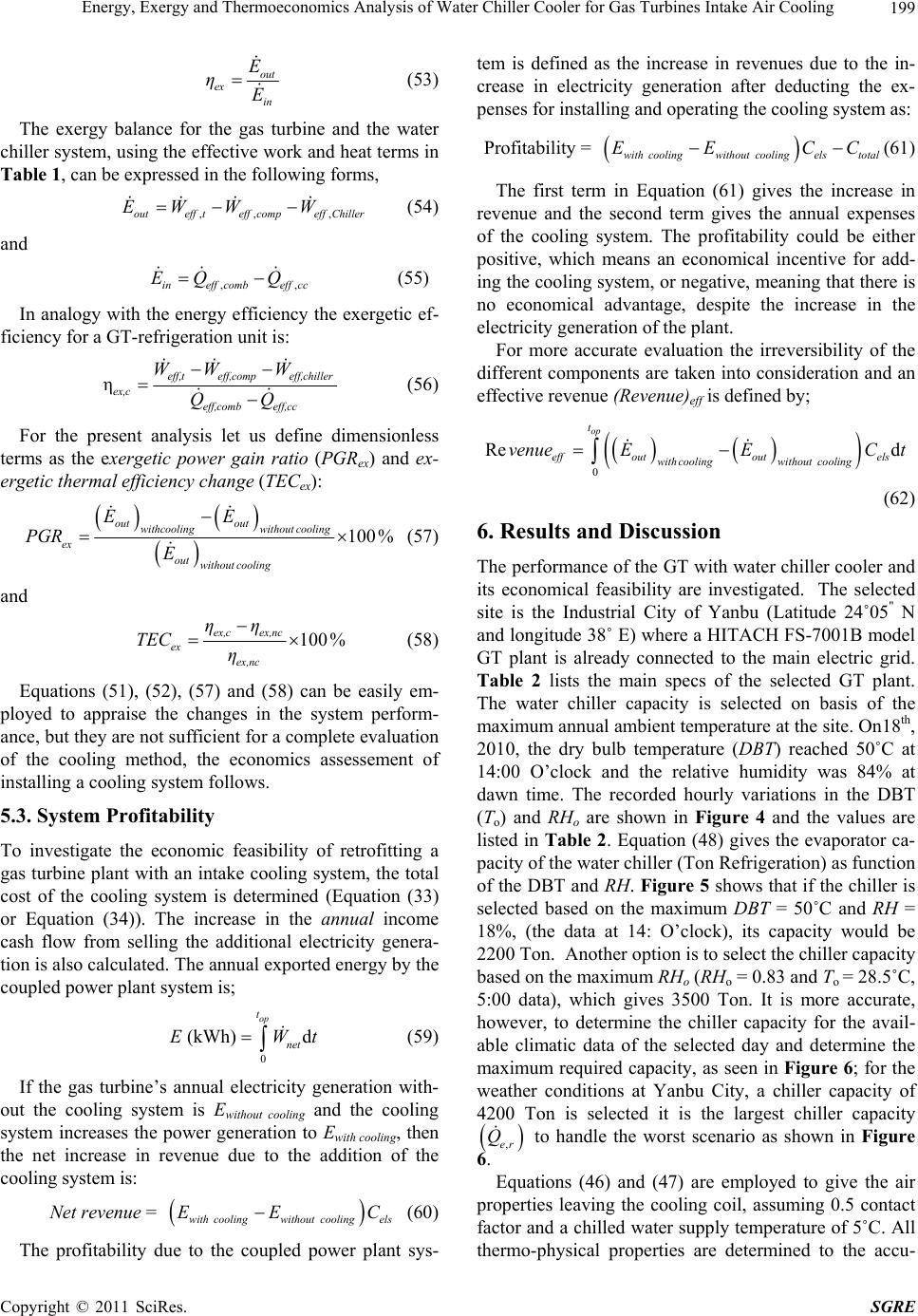 Energy, Exergy and Thermoeconomics Analysis of Water Chiller Cooler for Gas Turbines Intake Air Cooling199 out ex in E ηE (53) The exergy balance for the gas tu chiller system, using the effec rbine and the water tive work and heat terms in Table 1, can be expressed in the following forms, ,, ,outeff teff compeff Chiller EW WW (54) and ,,ineff combeff cc EQ Q (55) In analogy with the energy efficiency the exergetic ef- ficiency for a GT-refrigeration unit is: ηeff,t eff,comp eff,chiller ex,c eff,comb eff,cc W W W QQ (56) For the present analysis let us define dimensionless terms as the exergetic power gain ratio (PGRex) and ex- ergetic thermal efficiency change (TECex): 100% out out withcoolingwithout cooling ex out withoutcooling EE PGR E (57) and 100% ex,cex,nc ex ex,nc ηη TEC η (58) g a co 5.3. System Profitability To investigate the economic feasibility of retrofitting a ga ncome cash flow from selling the additional electricit tion is also calculated. The annual exported ener led power plant system is; generation with- out the cooling system is Ewithout cooling and the cooling system increases the power generation to E the net increase in revenue due to the addition of the co The profitability due to the coupled power plant sys- tem is defined as the increase in revenues due to the in- crease in electricity generation after deducting th penses for installing and operating the cooling system as: ability = Equations (51), (52), (57) and (58) can be easily em- ployed to appraise the changes in the system perform- ance, but they are not sufficient for a complete evaluation of the cooling method, the economics assessement of installinoling system follows. s turbine plant with an intake cooling system, the total cost of the cooling system is determined (Equation (33) or Equation (34)). The increase in the annual i y genera- gy by the coup 0 net If the gas turbine’s annual electricity (kWh) d op t EW t (59) with cooling, then oling system is: Net revenue = with coolingwithout co EE C (60) oling els e ex- Profit with coolingwithout coolingelstotal EE CC (61) The first term in Equation (61) gives the revenue and the second term gives the annual expenses of ersibility of the n into consideration and an The performance of the GT with water chiller cooler and its economical feasibility are investigated site is the Industrial City of Yanbu (Latitude 24˚05" N an mbient temperature at the site. On18, 2010, temperature (DBT) reached 50 14:00he relative humidity was 84 da sn the maximum DBT = 50˚C and R 18%, (the data at 14: O’clock), its capacity would be 22 a chiller capacity of 42 increase in the cooling system. The profitability could be either positive, which means an economical incentive for add- ing the cooling system, or negative, meaning that there is no economical advantage, despite the increase in the electricity generation of the plant. e irrevFor more accurate evaluation th different components are take effective revenue (Revenue)eff is defined by; 0 Re d op t eff outoutels with coolingwithoutcooling venueEEC t (62) 6. Results and Discussion . The selected d longitude 38˚ E) where a HITACH FS-7001B model GT plant is already connected to the main electric grid. Table 2 lists the main specs of the selected GT plant. The water chiller capacity is selected on basis of the maximum annual ath he dry bulb t˚C at O’clock and t% at wn time. The recorded hourly variations in the DBT (To) and RHo are shown in Figure 4 and the values are listed in Table 2. Equation (48) gives the evaporator ca- pacity of the water chiller (Ton Refrigeration) as function of the DBT and RH. Figure 5 shows that if the chiller is elected based oH = 00 Ton. Another option is to select the chiller capacity based on the maximum RHo (RHo = 0.83 and To = 28.5˚C, 5:00 data), which gives 3500 Ton. It is more accurate, however, to determine the chiller capacity for the avail- able climatic data of the selected day and determine the maximum required capacity, as seen in Figure 6; for the weather conditions at Yanbu City, 00 Ton is selected it is the largest chiller capacity ,er Q to handle the worst scenario as shown in Figure 6. Equations (46) and (47) are employed to give the air properties leaving the cooling coil, assuming 0.5 contac factor and a chilled water supply temperature of 5˚C. All thermo-physical properties are determined to the accu- t Copyright © 2011 SciRes. SGRE
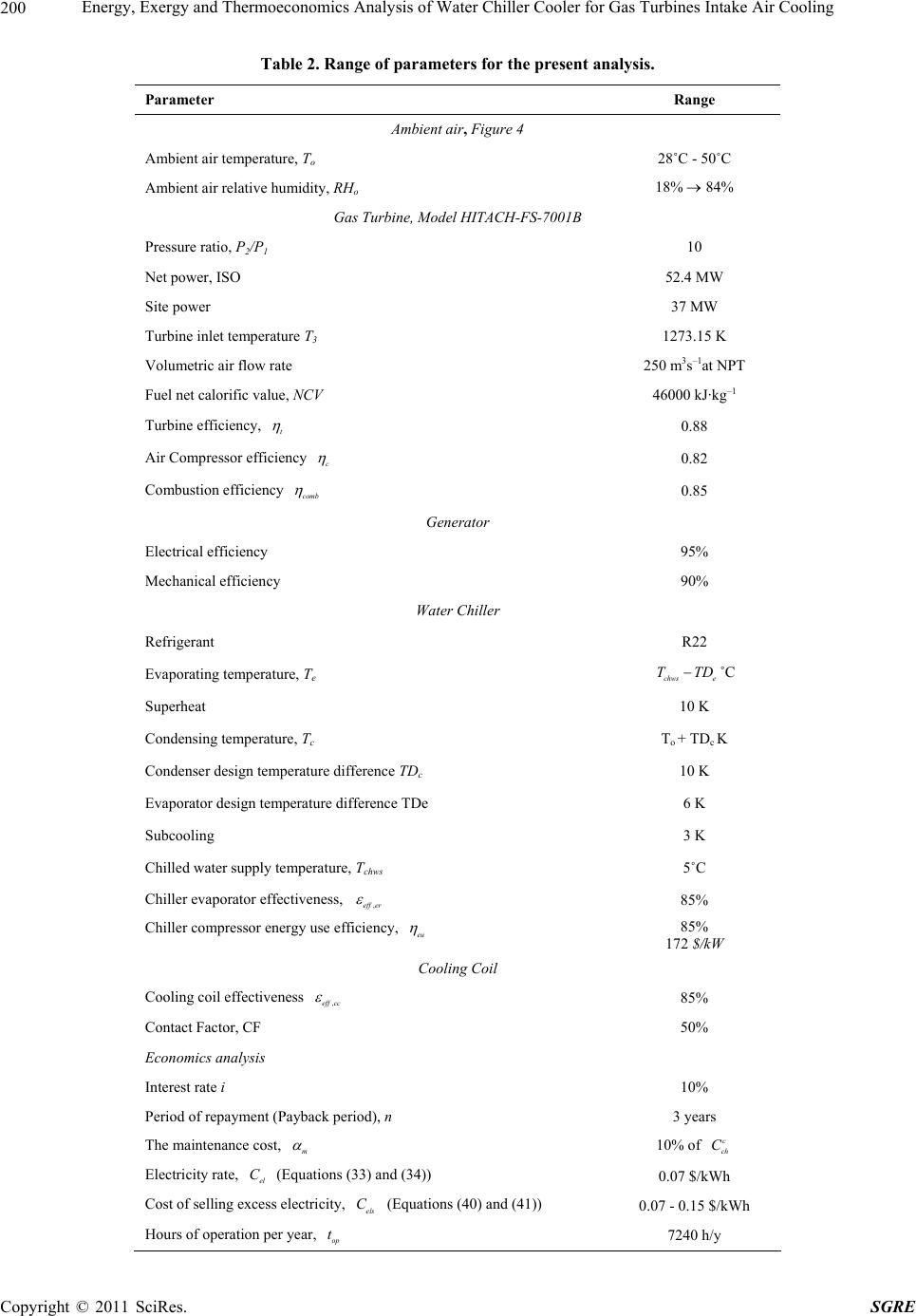 Energy, Exergy and Thermoeconomics Analysis of Water Chiller Cooler for Gas Turbines Intake Air Cooling Copyright © 2011 SciRes. SGRE 200 Table 2. Range of ptersarame for the present analysis. Range Parameter Ambient aFigure 4 ir, Ambient air temperature, To 28˚C - 50˚C Ambient air relative humidity, RHo 18% 84% Gas Turbine, ModeTACH-FS-7001B Pressure ratio, P2/P1 10 Net power, ISO 52.4 MW Site power 37 MW Turbine inlet temperature T3 Volumetric air flow rate Fuel net calorific value, NCV Turbine efficiency, t l HI 1273.15 K 250 m3s–1at NPT 46000 kJ·kg–1 Air Compressor efficiency 0.88 c 0.82 Combustion efficiency 0.85 Generator erature, Te ature difference TDc 10 ference TDe ate, Tchws 5 tiven s, Chiller compressor energy use efficiency, comb Electrical efficiency Mechanical efficiency 95% 90% Water Chiller Refrigerant R22 Evaporating tempchws e TD˚C T Superheat 10 K Condensing temperature, Tc T o + TDc K Condenser design temperK Evaporator design temperature dif6 K Subcooling 3 K Chilled water supply temperur˚C Chiller evaporator effec es,effer 85% eu 85% 172 $/kW Cooling Coil ss Contact Factor, CF 50% nalysis 10% payment (Payback period), n 3 Cooling coil effectivene,effcc 85% Economics a Interest rate i Period of reyears The maintenance cost, m 10% of c ch C Electricity rate, el C (Equations (33) and (34)) 0.07 $/kW ns (40) and (41)) 0.07 - 0.15 $/kWh ration per year, 7240 h/y h Cost of selling excess electricity, els C (Equatio Hours of opeop t
 Energy, Exergy and Thermoeconomics Analysis of Water Chiller Cooler for Gas Turbines Intake Air Cooling201 Figure 4. Ambient tempth of August 2010 of Yan erature variation and RH for 18 bu Industrial City. 20253035 404550 55 60 0 2000 4000 6000 8000 10000 12000 14000 16000 18000 T a o [C ] Chiller Cooling Capacity [TR] RH 100% 80% 60 % 40 % 20 % Figure 5. Dependence of chiller cooling capacity on the cli- matic conditions. = 0 2 4 6 810 1214 16 18 20 22 24 0 500 1000 1500 2000 2500 3000 3500 4000 4500 5000 hour [hr] Chiller Capacity [TR] 4204 Figure 6. Chiller capacity variation with the climatic condi- tions of the selected design day. show that the cooling system decrease e intake air temperature from To to T1 and increases the relative humidity to RH1 (Table 3). Solution of Equations 51 and 52, using the data in Ta- ble 3, gives the daily variation in PGR and TEC, Figure 7. There is certainly a potential benefit of adding the Table 3. The ambient cond and the cooling coil outlet temperature and humidity durth August 2010 opera- tion. Hour To˚C T1˚C RH1 racy of the Engineering Equation Solver (EES) software 36]. The result [ th itions ing 18 RHo 0 33.4 0.38 19.2 0.64 1 32.6 18.8 0.70 2 31.7 18.35 0.99 3 30.5 0.77 17.75 0.98 4 29.0 17.0 0.99 5 28.5 16.75 0.97 6 30.0 17.5 0.99 32.2 18.6 0.96 8 35.1 20.05 0.99 9 38.0 0.51 21.5 0.84 10 40.2 0.35 22.6 0.64 11 43.3 0.37 24.15 0.69 12 44.0 0.33 24.5 0.64 13 45.2 0.34 25.1 0.66 14 50.0 0.18 27.5 0.43 15 47.0 0.25 26.0 0.53 16 45.9 0.30 25.45 0.61 17 43.0 0.37 24.0 0.69 18 43.0 0.24 24.0 0.50 20 37.4 0.40 21.2 0.69 20.90 0.58 0.44 0.8 0.76 0.84 0.83 7 0.79 0.67 19 37.9 0.45 21.45 0.76 21 37.6 0.33 21.3 0.60 22 37.1 0.34 21.05 0.61 23 36.8 0.32 0246810 1214 16 18 20 22 24 0 2 4 6 8 10 12 14 16 18 -1 -0.5 0 0.5 1 1.5 2 hou PG R TEC [%] PGR [%] ] Figur. Variat gas tuPGR EC during 18th August operation. r [hr] [%] TEC [% e 7ion ofrbine and T Copyright © 2011 SciRes. SGRE
 Energy, Exergy and Thermoeconomics Analysis of Water Chiller Cooler for Gas Turbines Intake Air Cooling 202 cooling systemre the the power output all the tihe design day is 12.25%.PGR e pattern of the ambient temperature; the ner of plant reaches a mum .46%,lege in the plant therme pe appliion inds thataximucreasehe thermefficiechangonly 0.391% ocat 13:00 PM when the air temperature is 45.2˚C, and 34% RH. On basis of tcond lnalysisxergetw- er ga PGR is still itive meang that th increase in output a reduced value than that of the energy analysis. Figure 8 shohat thincrease for the worst day of the year that varies between 7% to 10.4% (average 8.5%d the tal effiy drop maxi of 6%. se resucateimportof the second law analysis. Ba on the variof the ent cons on Ast 18th ming rent vor selhe electricity (Cels)ation ves turly res needo paybnvnt afteecifiedra- tion peod (sel 3 y. The t term Equas (50)60) alculat prese in Figure 9. The effect of thmate changes is quite ob- ious on both the total expenses (Figure 9) and the GT net power output (Figure 7). The variations in are due to the changes in n Equation (50) thnds on ( where is an increase in me, the calculated ave The rage for t samefollows th increase i powthe GT maxi al efficien of 15 cy. Th with a litt ractical illu chan strativ caticate a mm de in t al ncy e of curs he seaw a the eic po in ratioex power but pos at inere is ws te power ) anhermciencs by amum Thelts indi the ance sed dailyation ambinditio ugu, assudiffealues fling t , Equ(60) gihe hoevenu ed t ri ack the i ected by estme ears) r a sp differen ope s in tion and (re caed andnted e cli v total C at depe ev Q i 1 ,, oo TT and 1 ). Th e po e ing e revenue from sel- tional electricity is also presented in the sam figure, wh shows clearly thtential of adding thling syste indicates that selling the ers at the sam base price (0.07 $/khsystem barl The profit increases directly with the cost of selling the elec- tricity. This result is interesting and encourages the utili- ties to consider a time-of-use tariff during the high de- mand periods. The profitability of the system, being th . The values show that there is al the base tariff. ling addi e e coo electricity to el = table. ich m. Figure 9 the consum Wh) makes t els CC ey profie cool e difference between the revenues and the total cost, is appreciable when the selling rate of the excess electricity generation is higher than the base rate of 0.07 $/kWh. Economy calculations for one year with 7240 opera- tion hours and for different electricity selling rates are summarized in Table 4 ways a net positive profit starting after the payback period for different energy selling prices. During the first 3 years of the cooling system life, there is a net profit when the electricity selling rate increases to 0.15 $/kWh, nearly double Figure 10 shows the effect of irreversibilities on the economic feasibility of using an air cooling system for the selected case. The effective revenue Equation (62) 0 2 4 6 810 121416 18 6 0 2 4 20 2224 8 10 12 14 16 18 -8.0 -6.0 -4.0 -2.0 0.0 2.0 h our [hr] PGR ex % TEC ex % TECex PGRex Figure 8. Variation of gas turbine exergetic PGRex and TE- Cex during 18th August operation. 0246810 1214 16 182022 2 0 200 400 600 800 1000 1200 hou Hourly To tal Cost C el s = 0.0 7 C el s = 0.10 Revenue r [hr ] [$], Revenu e [$] C el s = 0.15 [$/kW h] Total Cost profitability Fi to aT, HITACHI FS-7001B at Yanbu for different product rating Annual net profit for the first 3 years Annual net profit for the fourth year gure 9. Variation of hourly total cost and excess revenue at different electri ci ty selling rate. Table 4. Annual net profits out of retrofitting a cooling system tariff and 3 years pay bac k period. Electricity selling rate els C Annuity-for Chiller, coil and maintenance Annual ope cost G $/kWh $/y $/y $/y $/y 0.07 1,154,780 1,835,038 –1,013,600 +141180 0.1 1,154,780 1,835,038 –166,821 + 987,962 38 1,244,978 +2,399,758 0.15 1,154,780 1,835,0 C opyright © 2011 SciRes. SGRE
 Energy, Exergy and Thermoeconomics Analysis of Water Chiller Cooler for Gas Turbines Intake Air Cooling203 0 2 4 6 810 1214 16182022 2 0 100 200 300 400 500 600 Revenue ($/h) Revenue Revenue eff hour [hr] Eq. 62Eq. 62 Eq. 60Eq. 60 Figure 10. Effect of irreversibility on the revenue, Cels = 0.07 ulated from selling the ed l versibilities. The major contres from the w ter chille irreversibility is the highest. 7. Conclusions There arerious methods to the perform of gas turbine power plants operating under hot ambient mperatures far from the ISO standards. One pr pproach is to reduce the compressor intake temperature by installing an external cooling system. In this paper, a simulation model that consists of thermal analysis of a GT and coupled to a refrigeration cooler, exergy analysis and economics evaluation is developed. The performed analysis is based on coupling the thermodynamics pa- rameters of the GT and cooler unit with the other vari- ables as the interest rate, life time, increased revenue and profitability in a single cost function. The augmentation of the GT plant performance is characterized using the power gain ratio (PGR) and the thermal efficiency change term (TEC). The developed model is applied to a GT power plant (HITACHI FS-7001B) in the city of Yanbu (20˚05" N s reached 50˚C on August 18, 2010. The re- d climate conditions on that day are selected for sizing out th rease output power is 12.25%, with insig- plant thermal efficiency. The second between 0.07 and 0.15 $/kWh and a payback period of 3 years. Cash flow analysis of the GT power plant in the city of Yanbu shows a potential for increasing the output power of the plant and increased revenues. REFERENCES [1] Cortes CPE, D. Williams, “Gas Turbine Inlet Cooling Techniques: An Overview of Current Technology,” Pro- ceedings Power GEN 2003, Las Vegas, 9-11 December 2003. [2] T, Wang, X. Li and V. Pinniti, “Simulation of Mist Transport for Gas Turbine Inlet Air-Cooling,” ASME In- ternational Mechanical Engineering Congress, Anaheim Keshtgar, “Gas Turbine ing Systems Design And Analysis, Manchester, 2004. ian a- parison oe Inlet Air Coos to En- hance the Gas Turbine Generated Poweternational Journal of En, Vol. 31, 2007. 483-503. doi:10. 5 $/kWh. Re eff venue that can be accum Power Augmentation Using Fog Inlet Cooling System,” Proceedings ESDA04 7th Biennial Conference Engineer- net power output is ruced by 41.8% as a resu ibution com t of irre- a- [4] er, where th va improveance te a oven latitude and 38˚E longitude) KSA, where the maximum DBT hath corde e chiller and cooling coil capacities. The performance analysis of the GT shows that the intake air temperature decreases by 12 to 22 K, while the PGR in- creases to a maximum of 15.46%. The average inc in the plant power nificant change in law analysis show that the exergetic power gain ratio drops to an average of 8.5% with 6% maximum decrease in thermal efficiency. In the present study, the profitability resulting from cooling the intake air is calculated for electricity rates , 13-19 November 2009. [3] M. Ameri, H. Nabati and A. M. Ameri, H. R. Shahbaz f Evaporativ nd M. Nabizadeh, “Com ling System r,” In , ppergy Research 1002/er.131 [5] M. Jonsson and J. Yan, “Humidifirbines-A Review of Implemented CEnergy, Vol. 30. doi:10.1016/j.energy.2004.08.005 ed Gas Tu ycles,” Proposed and , 2005, pp. 1013-1078 [6] M. M. Alhazmy and Y. S. Najjar, “Augmentation of Gas Turbine Performance Using Air Coolers,” Applied Ther- mal Engineering, Vol. 24, 2004, pp. 415-429. doi:10.1016/j.applthermaleng.2003.09.006 [7] M. M. Alhazmy, R. K. Jassim and G. M. Zaki, “Per- formance Enhancement of Gas Turbines by Inlet Air-Cooling in Hot and Humid Climates,” International Journal of Energy Research, Vol. 30, 2006, pp. 777-797. doi:10.1002/er.1184 [8] S. Sanaye and M. Tahani, “Analysis of Gas Turbine Op- erating Parameters with Inlet Fogging and Wet Compres- sion Processes,” Applied Thermal Engineering, Vol. 30, 2010, pp. 234-244. doi:10.1016/j.applthermaleng.2009.08.011 [9] T. C. Tillman, D. W. Blacklund and J. D. Penton, “Ana- lyzing the Potential for Condensate Carry-Over from a Gas Cooling Turbine Inlet Cooling Coil,” ASHRAE Transactions, Vol. 111, 2005, pp. 555-563. [10] M. Chaker, C. B. Meher-Homji and M. Mee, “Inlet Fog- ging of Gas Turbine Engines—Part B: Fog Droplet Sizing Analysis, Nozzle Types, Measurement and Testing,” ASME Proceedings of Turbo Expo 2002, Vol. 4, 2002. pp. 429-442. [11] M. Chaker, C. B. Meher-Homji and M. Mee, “Inlet Fog- ging of Gas Turbine Engines—Part C: Fog Behavior in Inlet Ducts, Cfd Analysis and Wind Tunnel Experi- ments,” ASME Proceedings of Turbo Expo 2002, Vol. 4, 2002, pp. 443-455. [12] M. Chaker, C. B. Meher-Homji, Mee III and A. Nichol- Copyright © 2011 SciRes. SGRE
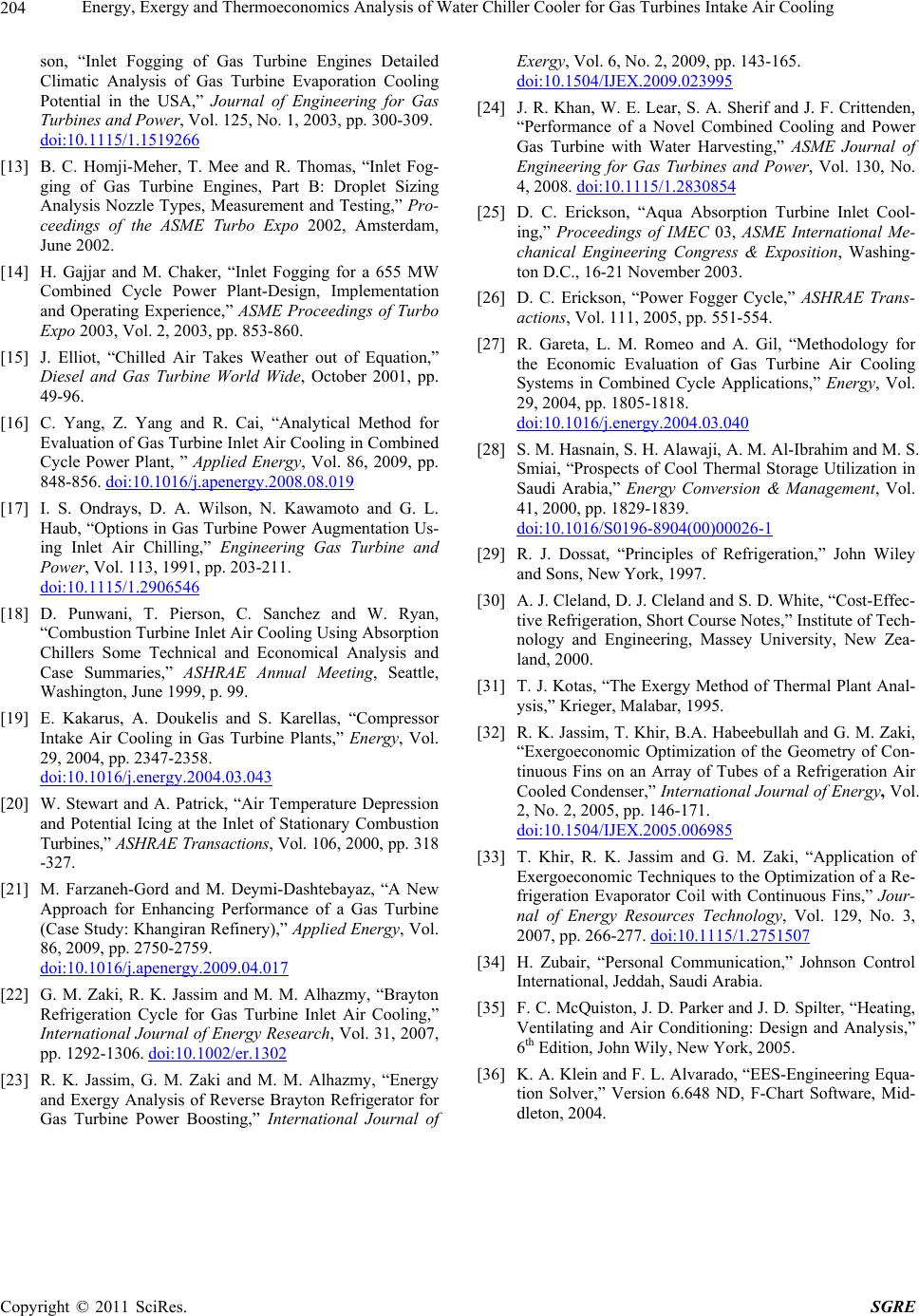 Energy, Exergy and Thermoeconomics Analysis of Water Chiller Cooler for Gas Turbines Intake Air Cooling 204 son, “Inlet Fogging of Gas Turbine Engines Detailed Climatic Analysis of Gas Turbine Evaporation Cooling Potential in the USA,” Journal of Engineering for Gas Turbines and Power, Vol. 125, No. 1, 2003, pp. 300-309. doi:10.1115/1. 1519266 eher, T. Mee and R. T[13] B. C. Homji-Mhomas, “Inlet Fog- 02. pp. 853-860. for ging of Gas Turbine Engines, Part B: Droplet Sizing Analysis Nozzle Types, Measurement and Testing,” Pro- ceedings of the ASME Turbo Expo 2002, Amsterdam, June 20 [14] H. Gajjar and M. Chaker, “Inlet Fogging for a 655 MW Combined Cycle Power Plant-Design, Implementation and Operating Experience,” ASME Proceedings of Turbo Expo 2003, Vol. 2, 2003, [15] J. Elliot, “Chilled Air Takes Weather out of Equation,” Diesel and Gas Turbine World Wide, October 2001, pp. 49-96. [16] C. Yang, Z. Yang and R. Cai, “Analytical Method Evaluation of Gas Turbine Inlet Air Cooling in Combined Cycle Power Plant, ” Applied Energy, Vol. 86, 2009, pp. 848-856. doi:10.1016/j.apenergy.2008.08.019 [17] I. S. Ondrays, D. A. Wilson, N. Kawamoto and G. L. Haub, “Options in Gas Turbine Power Augmentation Us- ing Inlet Air Chilling,” Engineering Gas Turbine and Power, Vol. 113, 1991, pp. 203-211. doi:10.1115/1.2906546 [18] D. Punwani, T. Pierson, C. Sanchez and W. Ryan, , Seattle, “Combustion Turbine Inlet Air Cooling Using Absorption Chillers Some Technical and Economical Analysis and Case Summaries,” ASHRAE Annual Meeting Washington, June 1999, p. 99. [19] E. Kakarus, A. Doukelis and S. Karellas, “Compressor Intake Air Cooling in Gas Turbine Plants,” Energy, Vol. 29, 2004, pp. 2347-2358. doi:10.1016/j.energy.2004.03.043 [20] W. Stewart and A. Patrick, “Air Temperature Depression yaz, “A New and Potential Icing at the Inlet of Stationary Combustion Turbines,” ASHRAE Transactions, Vol. 106, 2000, pp. 318 -327. [21] M. Farzaneh-Gord and M. Deymi-Dashteba Approach for Enhancing Performance of a Gas Turbine (Case Study: Khangiran Refinery),” Applied Energy, Vol. 86, 2009, pp. 2750-2759. doi:10.1016/j.apenergy.2009.04.017 [22] G. M. Zaki, R. K. Jassim and M. M. Alhazmy, “Brayton Refrigeration Cycle for Gas Turbine Inlet Air Cooling,” International Journal of Energy Research, Vol. 31, 2007, pp. 1292-1306. doi:10.1002/er.1302 [23] R. K. Jassim, G. M. Zaki and M. M. Alhazmy, “Energy and Exergy Analysis of Reverse Brayton Refrigerator for Gas Turbine Power Boosting,” International Journal of Exergy, Vol. 6, No. 2, 2009, pp. 143-165. doi:10.1504/IJEX.2009.023995 [24] J. R. Khan, W. E. Lear, S. A. Sherif and J. F. Crittenden, “Performance of a Novel Combined Cooling and Power Gas Turbine with Water Harvesting,” ASME Journal of Engineering for Gas Turbines and Power, Vol. 130, No. 4, 2008. doi:10.1115/1.2830854 [25] D. C. Erickson, “Aqua Absorption Turbine Inlet Cool- ing,” Proceedings of IMEC 03, ASME International Me- n Combined Cycle Applications,” Energy, Vol. chanical Engineering Congress & Exposition, Washing- ton D.C., 16-21 November 2003. [26] D. C. Erickson, “Power Fogger Cycle,” ASHRAE Trans- actions, Vol. 111, 2005, pp. 551-554. [27] R. Gareta, L. M. Romeo and A. Gil, “Methodology for the Economic Evaluation of Gas Turbine Air Cooling Systems i 29, 2004, pp. 1805-1818. doi:10.1016/j.energy.2004.03.040 [28] S. M. Hasnain, S. H. Alawaji, A. M. Al-Ibrahim and M. S. Smiai, “Prospects of Cool Thermal Storage Utilization in Saudi Arabia,” Energy Conversion & Management, Vol. 41, 2000, pp. 1829-1839. doi:10.1016/S0196-8904(00)00026-1 [29] R. J. Dossat, “Principles of Refrigera and Sons, New York, 19 tion,” John Wiley 97. the Geometry of Con- [30] A. J. Cleland, D. J. Cleland and S. D. White, “Cost-Effec- tive Refrigeration, Short Course Notes,” Institute of Tech- nology and Engineering, Massey University, New Zea- land, 2000. [31] T. J. Kotas, “The Exergy Method of Thermal Plant Anal- ysis,” Krieger, Malabar, 1995. [32] R. K. Jassim, T. Khir, B.A. Habeebullah and G. M. Zaki, “Exergoeconomic Optimization of tinuous Fins on an Array of Tubes of a Refrigeration Air Cooled Condenser,” International Journal of Energy, Vol. 2, No. 2, 2005, pp. 146-171. doi:10.1504/IJEX.2005.006985 [33] T. Khir, R. K. Jassim and G. M. Zaki, “Application of Exergoeconomic Techniques to the Optimization of a Re- frigeration Evaporator Coil with Continuous Fins,” Jour- nal of Energy Resources Technology, Vol. 129, No. 3, 2007, pp. 266-277. doi:10.1115/1.2751507 [34] H. Zubair, “Personal Communication,” Johnson Control International, Jeddah, Saudi Arabia. [35] F. C. McQuiston, J. D. Parker and J. D. Spilter, “Heating, Ventilating and Air Conditioning: Design and Analysis,” th 6 Edition, John Wily, New York, 2005. [36] K. A. Klein and F. L. Alvarado, “EES-Engineering Equa- tion Solver,” Version 6.648 ND, F-Chart Software, Mid- dleton, 2004. C opyright © 2011 SciRes. SGRE
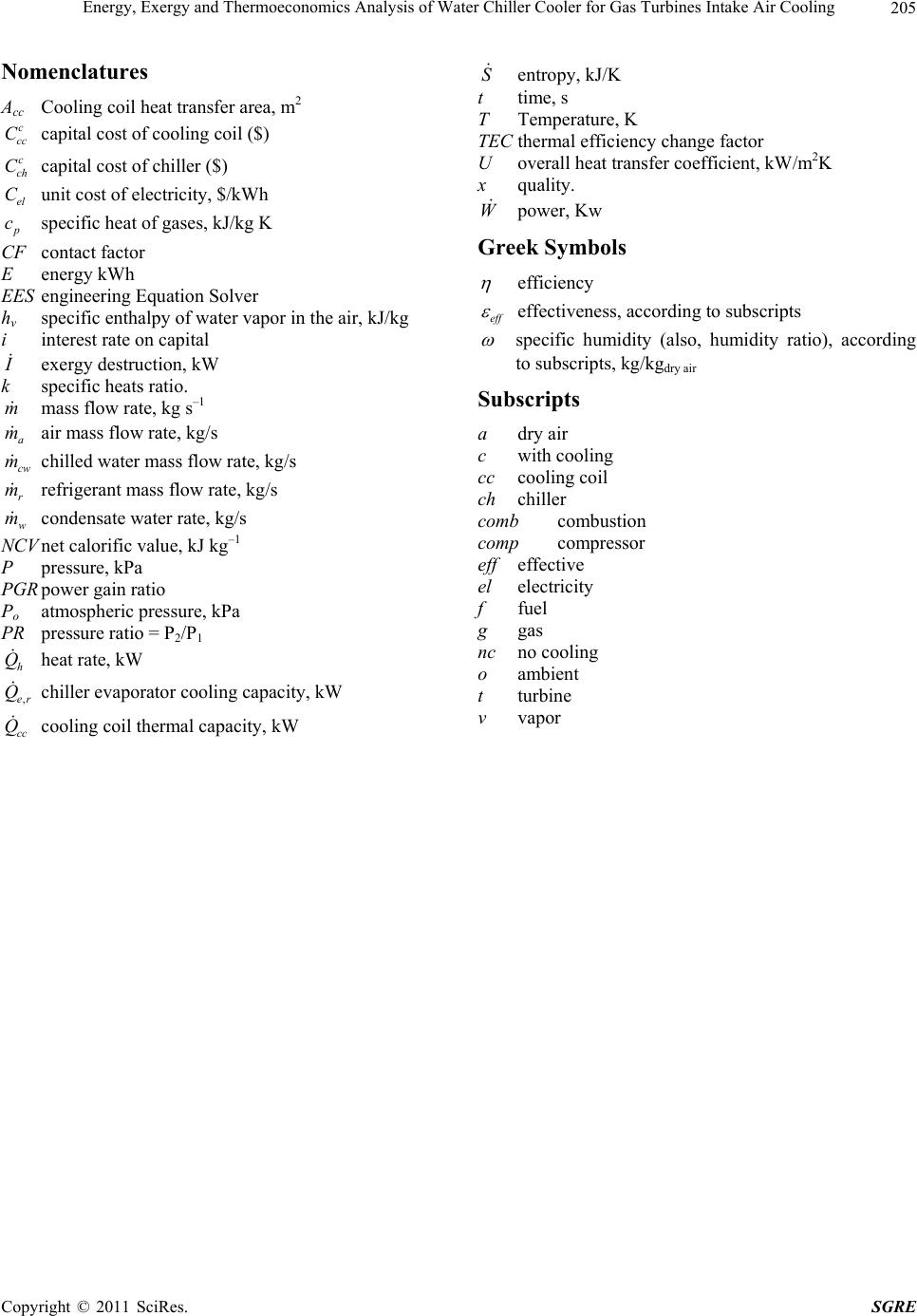 Energy, Exergy and Thermoeconomics Analysis of Water Chiller Cooler for Gas Turbines Intake Air Cooling205 Nomenclatures Acc Cooling coil heat transfer area, m2 c cc C capital cost of cooling coil ($) c ch C capital cost of chiller ($) el C unit cost of electricity, $/kWh c specific heat of gases, kJ/kg K CF contact factor E energy kWh EES engineering Equation Solver hv specific enthalpy of water vapor in the air, kJ/kg i interest rate on capital exergy destruction, kW k specific heats ratio. m mass flow rate, kg s–1 m air mass flow rate, kg/s a cw m chilled water mass flow rate, kg/s r m refrigerant mass flow rate, kg/s condensate w m water rate, kg/s NCV net calorific value, kJ kg–1 P pressure, kPa PGR power gain ratio Po atmospheric pressure, kPa PR pressure ratio = P2/P1 heat rate, kW chiller evaporator cooling capacity, kW cooling coil thermal capacity, kW h Q ,er Q cc Q S entropy, kJ/K t time, s T Temperature, K TEC thermal efficiency change factor U overall heat transfer coefficient, kW/m2K x quality. W power, Kw Greek Symbols efficiency eff effectiveness, according to subscripts specific humidity (also, humidity ratio), according to subscripts, kg/kgdry air Subscripts dry air a c with cooling cc cooling coil ch chiller comb combustion comp compressor eff effective el electricity f fuel g gas nc no cooling o ambient t turbine v vapor Copyright © 2011 SciRes. SGRE
|