 Materials Sciences and Applications, 2015, 6, 963-977 Published Online November 2015 in SciRes. http://www.scirp.org/journal/msa http://dx.doi.org/10.4236/msa.2015.611097 How to cite this paper: Van Khai, T., Lam, T.D., Van Thu, L. and Kim, H.W. (2015) A Two-Step Method for the Preparation of Highly Conductive Graphene Film and Its Gas-Sensing Property. Materials Sciences and Applications, 6, 963-977. http://dx.doi.org/10.4236/msa.2015.611097 A Two-Step Method for the Preparation of Highly Conductive Graphene Film and Its Gas-Sensing Property Tran Van Khai1*, Tran Dai Lam2, Le Van Thu3, Hyoun Woo Kim4 1Faculty of Materials Technology, Ho Chi Minh City University of Technology, Ho Chi Minh City, Vietnam 2Institute of Material Science, Vietnam Academy of Science and Technology, Hanoi, Vietnam 3Institute of Chemistry-Biology and Professional Documents, Ministry of Public Security, Hanoi, Vietnam 4Division of Materials Science and Engineering, Hanyang University, Seoul, Republic of Korea Received 24 September 2015; accepted 15 November 2015; published 18 November 2015 Copyright © 2015 by authors and Scientific Research Publishing Inc. This work is licensed under the Creative Commons Attribution International License (CC BY). http://creativecommons.org/licenses/by/4.0/ Abstract In this research, a highly conductive graphene film was synthesized through the chemical reduc- tion of graphene oxide (RGO) nanosheets followed by thermal treatment at 1100˚C (RGO-1 100˚C) under H2 ambient. The as-prepared graphene films were characterized by using X-ray photoelec- tron spectroscopy, fourier transform infrared spectroscopy, X-ray diffractions, raman spectros- copy, transmission electron microscopy, scanning electron microscopy, atomic force microscopy and by electrical conductivity measurements. The results showed that the thermal treatment effi- ciently removed residual oxygen-containing functional groups on the surface of the RGO sheets and simultaneously restored the sp2 carbon networks in the graphene sheets. As a result, the elec- trical conductivity of RGO-110 0˚C (~210 S/cm) film was greatly improved compared with that of RGO (~24 S/cm) and graphene oxide (4.2 × 10−4 S/m) films. In addition, the NO2 gas sensing cha- racteristics of the as-prepared RGO films were studied. The results indicated that RGO films were highly responsive to NO2 at temperature of 200˚C. Keywords Graphene, Graphene Oxide, Graphene-F ilm, Thermal Reduction 1. Introduction Graphene, a single layer of sp2-hybridized carbon atoms in a hexagonal two-dimensional lattice, has attracted
 T. Van Khai et al. tremendous attention due to its unique ph ys ic a l a nd chemical prop e rties and potential applications in many fields, including nanoelectronic, sensor for biomolecules, transistors, solar cell and catalysts [1]-[4]. Up to now, there are major methods having been developed to synthesize graphene, including mechanical exfoliation, epitaxial growth, bottom-up synthesis and chemical reduction of graphene oxide suspension. The last method is consi- dered to an efficient approach to produce graphene sheets in bulk quantity at relatively low cost. Currently, the Hummers’ method is the most widely used technique for preparing graphene oxide (GO) [5], whic h invol ves oxid atio n of grap hite in the prese nce of str ong aci ds and o xidant s. When oxidi zed, GO still p os- sess a layered structure, being composed of unoxidized aromatic regions and aliphatic regions, which contain many oxygen functional groups [6] [7]. The π-conjugated system in graphene is disrupted by these oxygen- containing functional groups, producing separated nanocrystalline graphene. Since the as-prepared GO is an electrical in sulator, various reduction methods have been developed to efficiently recover its electrical property [8]-[11]. Chemical reduc tion of GO i s one o f the most pr omisin g method s to realize lo w-cost and high-through- put preparation of converted graphene with excellent process ability [12]. However, the chemically RGO sheets still exhibit much lower conductivity than pristine graphene, mainly due to the presence of irreversible defects, disorder and residual functional groups. Therefore, it is necessary to develop a more effective reduction method to produce graphene-like film with high electrical conductivity. Recently, it is demonstrated that thermal an- nealing is the most effective method capable of removing oxygen from GO films, where reduction is accom- plished by decomposition of oxygen containing groups and simultaneous restoration of sp2 carbon networks [13]. Hence, high-temperature annealing is required to repair the defects and further remove the residual functional groups in RGO sheets to improve its electrical properties. Bec ause t he grap he ne fi lm po sses ses na no metri c thic kne ss, high electrical conductivity, high aspect ratio, and good mechanical property; it has been regarded as a promising material for gas sensor devices. Recent studies on the interaction of graphene with gas molecules have indicated that it can act as a good sensor [14]-[21]. Schedin and co-workers [19] have shown that the increase in the charge carrier concentration induced by gas molecules adsorbed on the surface of graphene can be used to fabricate sensitive gas sensors. Based on theoreti- cal investigations on the adsorption of gas molecules o n single-layer grap hene as well as on graphe ne nanorib- bons, it has been predicted that the doping in carbon nanostructures may improve the sensitivity [22]-[25]. Ao and co-workers have applied density functional theory to show that aluminium doped graphene can be used as a good detector for carbon monoxide [26]. Functionalized graphite nanostructures are able to sense traces of pol- lutant gases such as N O2 [27]. Water vapor sensing characteristics of RGO has been studied [28]. RGO is also shown to be good sensor for toxic vapors [29]. Spin-coated hydrazine functionalized graphene dispersions are able to detect NO2, NH3, and 2,4-dinitrotoluene [30]. However, very little work has reported about NO2 gas sensor based on graphene film prepared through thermal reduction of RGO films. In this paper, we employed a simple method for t he preparation of hig hly cond uctive graphene films thr ough the chemical reduction of GO and subsequent thermal annealing at 1100˚C in H2 ambient. We have studied the relation between structure and electrical conductivity of the graphene films upon reduction. Further, the NO2 gas sensors based on the RGO films have been fabricated onto the Pt electrodes on a quartz substrate and studied. It is considered to be important to examine gas sensing characteristics of graphene films prepared by chemical and thermal red uction. 2. Experiments 2.1. Materials Graphite powder (Qingdao Yanshou Graphite Co., Ltd. Qingdao Branch, China), H2SO 4 (98%, Viet Nam), H3PO4 (98%, Viet Nam), KMnO4 (98%, Japan) and H2O2 (30 wt%), Hydrazine monohydrate (98%, Otsuka Chemical Co., Ltd., Japan), N,N-Dimethylformamide (DMF, 99.8%, Otsuka Chemical Co., Ltd., Japan) were obtained from commercial resources and used as received. 2.2. Fabrication of Graphene Films and Sensor Device GO was synthesized from graphite powder via a modification of Hummers and Offeman’s method [5]. In a typ- ical reaction, 5 g of graphite, 60 mL of H3PO4, and 180 mL of H2SO4 were stirred together with a Teflon-coated magnetic stirri ng in an ice bat h. Next, 60 g of KM nO4 was slowly added while the temperature was maintained
 T. Van Khai et al. at 0˚C. Once mixed, the solution is transferred to a 35˚C ± 5˚C water bath and stirred for 3h, forming a thick paste. Next, distille d water (450 mL) was slowly dr opped into the resulting paste to dilute the mixture, and then the solution was stir red for 1 h while the temperature was raised to 9 0˚C ± 5˚C. Finally, 800 mL of distilled wa- ter was added, followed by the slow addition of 60 mL H2O2 (30%), turning the colour of the solution from dark brown to yello w. During this final step , H2O2 (30%) reduced the residual permanganate and manganese dioxide to colourle ss soluble mangane se sulphate. T he GO deposit was collected fro m the GO suspension by high speed centrifugation at 15,000 rpm for 30 min. The obtained GO was then washed with 1000 mL of HCl (5%), and repeatedly washed with distilled water until the pH = 7. To obtain uniform GO, a low-speed centrifugation at 3000 rp m was first used to remove thick multila yer sheets unt il all the visible par ticles were removed (3 - 5 min). Then the supernatant was further centrifuged at 10,000 rpm for 30 min to remove small GO pieces and water- soluble by product. The final sediment was redispersed in 500 ml of DMF with mild sonication, resulting in a solution of exfoliated GO. The exfoliated GO nanosheets were chemically reduced to graphene in the presence of hydrazine. Typical, 500 ml of above exfoliated GO was stirred for 30 min, and 10 ml of hydrazine monohy- drate was added. The mixtures were heated at 150˚C ± 5˚C using an oil bath for 24 h; a black solid precipitated (called RGO) from the reaction mixtures. Products were collected by centrifugation at 12,000 rpm for 45 mi- nutes and washed with deioniz ed wate r a nd methanol until the pH = 7. Ne xt, to generate a homo geno us colloidal suspension of RGO, the obtained RGO was redispersed in DMF, and then the mild sonication was applied for 60 minutes to form a homogenous RGO suspension. Subsequently, the RGO suspension was sprayed on quartz substr ate s using t he air-brush s ystem with Ar as a carrier gas. A horizontal quartz tube furnace was used for an- nealing RGO films at 1100˚C (RGO-1100˚C) under a mixture of argon (Ar) and hydrogen (H2) gas. The films were put on the top of the alumina boat is then introduced into the quart tube reactor filled with Ar + H2 gas (20% H2). To avo id b urning o f GO fil ms at hi gh te mper ature due t o the self-released oxygen from GO sheets, the quartz tube was evacuated to about 10−2 Torr using rotary vacuum pump. Next, the system is first heated for 180 min at 250˚C to remove all the moisture with (Ar + H2) gas flowing at a rate of 100 sccm. Subse- quently the temperature was raised to 1100˚C in 60 min. The annealing reaction was carried out for 30 min. Finally, the system was naturally cooled down to room temperature at constant (Ar + H2) flow rate of 100 sccm. To evaluate the sensing behavior of the RGO films, we have sprayed the RGO suspension onto the interd igi- tated Pt electrode on a quartz substrate, followed by thermal treatment with the same conditions as mentioned above. 2.3. Characterization of the As-Synthesized Materials The microstructures of the GO, RGO and as-synt hesize d fil ms were investigated by using scanning electron mi- croscope (SEM, JEOL, JSM-5900 LV, Japan). Atomic force microscope (AFM) image was obtained on an AFM XE-100 (Park system) equipped. High-resolution transmittance electron microscope (HR-TEM) was conducted on a TECNAI 20 microscope operated at 200 kV. X-ray diffraction (XRD) characterization was performed us- ing Cu Kα (wavelength at 1.54 Å) radiation, 40 kv, 30 mA, Rigaku, Japan. The Fourier transform infrared (FT-IR) spectra (500 - 4000 cm−1) were measured using a (Nicolet IR100 FT-IR) spectrometer with KBr (99%) as background. The Raman spectroscopy measurements were recorded using a Jasco Laser Raman Spectrophotometer NRS-3000 Series, with an excitation laser wavelength of 532 nm, at a power density of 2.9 mW ∙cm−2. X-ray photoelectron spectroscopy (XPS, VG Multilab ESCA 2000 system, UK) analysis using a monochromatized Al Kα X-ray source (hν = 1486.6 eV) was performed to analyze the elemental composi- tions and the assignments of the carbon peaks of the samples. The current-voltage (I-V) characteristic is ex- amine d b y us i ng four point probes with Keithley 2400 Source-meter. Preliminary current measurements in a ga s sensor characterization system (GSCS) were performed exposing the device. Sensing characteristics were eva- luated at various NO2 concentrations, in the ra nge of 10 - 50 ppm, at an operating temperature of 200˚C under atmospheric pressure. 3. Results and Discussion 3.1. Morphology and Stru ct u re Figure 1(a) & Figure 1(b) sho ws SEM image of graphite powers used as staring material with a wide size range of the particles, where the dimensions are about 3 - 7 μm in width and several hundreds of nm in thickness
 T. Van Khai et al. Figure 1 . SEM images of graphite powders (a) & (b) and of GO (c) & (d). (~7 50 nm) with fla ke-shape. Figure 1(c) & Figure 1(d) reveals SEM image of GO nanosheets. The result indi- cates that the average particle size dramatically reduces after the oxidation process. During the oxidation reac- tion of graphite powder with concentrated sulfuric acid and phosphoric acid, H2O molecules, and cations were inserted into the graphene layers and expanded the interlayer spacing in the graphite crystal struc- ture [7]. The graphite oxide can be effectively exfoliated via ultrasonic vibration to create GO nanosheets. Fur- ther observation of morpholo gy has been achieved tho ugh TEM. As shown in Figure 2(a), the transparent GO nanosheets exist in the form of thin few-layer grephene with folds and wrinkles. The edges of the GO nano- sheets are distinguishable, and found to contain a tri-layer GO fla ke, as s hown in the in set of Figure 2(a). Wrin- kles and folds are very evident on the surface o f the GO nanosheets; these are characteristic of thin and two-di- mensional (2D) GO. The AFM image (Figure 2( b)) also s hows a 2 D GO na nosheet with wri nkle-like features, and the apparent thickness is ca. 1.2 nm, which is comparable to the literature data (1.25 nm) for the bi-layer GO nanosheet as reported before [31]. Theoretically, the single-layer graphene sheet is atomically flat with a well- known van d er Waals thick ness of 0. 34 n m, while G O she et s are expected to be “thicker” due to the presence of covalently bound oxygen and the displacement of the sp3-hybrid ize d ca rbon a to ms sli ghtl y ab ove and b elo w the original graphene plane. Some researchers [32] pointed out that the heights of “dry” and hydrated GO were about 0.65 - 0.75 nm and 1.2 nm, respectively. Therefore, in this paper, the GO nanosheets may be composed of a bi- and/or tri-layer of G O sheets, being in good agre ement with the TEM r esult. Figure 3(a) displays a typical TEM image of RGO sheets. It is observed that the RGO sheet is made of 3-4 layers, a s sho wn in the botto m right -corner of Figure 3(a). T he increase in number of layers of RGO compared to GO can be due to aggregation of graphene sheets after chemical reduction process, similar to the previous studies [33] [34]. The crystallographic structure of the RGO is determined by using the selected area electron diffraction (SAED) technique, as shown in the inset of Figure 3(a). The SAED yields a ring-shaped pattern consisting of many diffraction spots for each order of diffraction. These spots make regular hexagons with dif- ferent rotational angles, indicating the presence of few layers in the RGO assembly. Furthermore, this result confirms that the typical six-fold symmetry of the pattern comes from the crystallographic structure of the gra- phene composed by carbon atoms arranged in a strictly two-di mens iona l ho ne ycomb—in real space. Figure 3(b) and Figure 3(c) show two-dimensional (2D) and three-dimensional (3D) AFM images of RGO-1100˚C films, respectively. AFM images of RGO-1100˚C films revealed that the RGO-1100˚C sheet sizes of a few microns
 T. Van Khai et al. Figure 2 . TEM image of GO (a) and AMF image of GO (b) and the height profile in selected location. Figure 3 . (a) TEM of RGO nanosheets. The inset in Figure 3(a) is corr espo ndi ng sel ected area ele ctr on dif- fraction (SAED); (b) Two-dimensional; and (c) three-dimensional AFM images of RGO-1100˚C films.
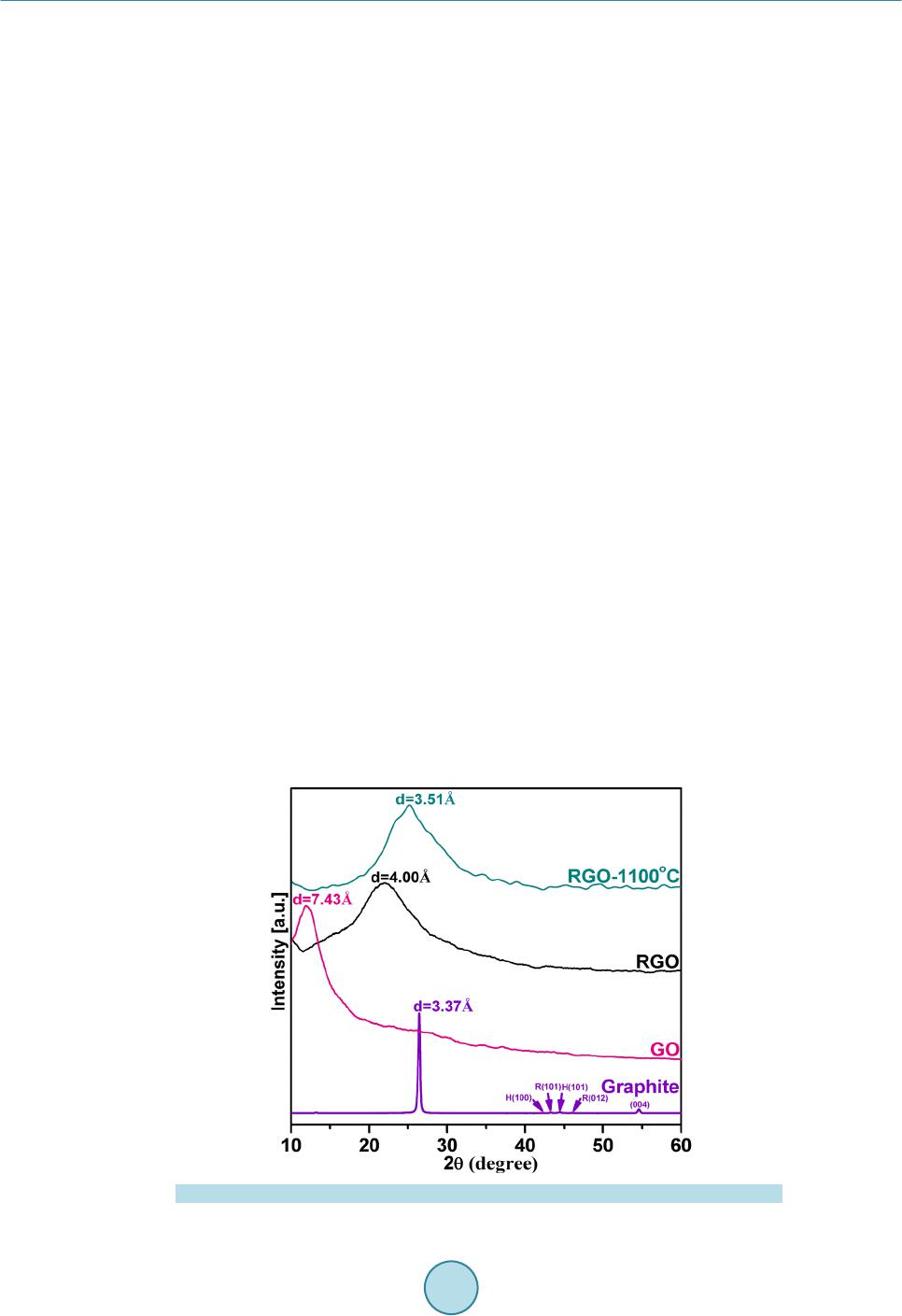 T. Van Khai et al. were randomly deposited an overlapped one another to create a continuous thin film with long, broad wrinkles or folds. In spite of the formation of the wrinkles and folds across the film surface, the surface root mean square roughness (Rq) was estimated to be about 0.965 nm, which is comparable to that of the reduced GO film pre- pared by the same method [35]. Figure 4 shows the XRD patterns of raw graphite, GO, RGO and RGO-1100˚C films. The raw graphite showed a very strong (002) peak at 26.40˚, corresponding to interlayer distance (d-spacing) of about 3.37 Å. However, after oxidation, the (002) peak shifted to a lower angle of around 11.90˚, corresponding to the d- spacing of 7.43 Å. Such d-spa ci ng is si gni fica ntl y lar ger tha n tha t of si ngl e-la yer gr ap hen e (~ 3.36 Å), indicating that GO contains large numbers of oxygen-containing functional groups on both sides of the graphene sheets. For RGO sample, the peak disappeared in a region of low angle and another broad peak at 22.19˚ corresponding to d-spacing of 4.0 Å appeared. This indicated that a large number of functional groups on the surface of GO was removed during chemical reduction process. As the RGO films was annealed at 1100˚C, the ( 002) pe ak be- came sharper and shifted to higher angle of 25.30˚. T he d -spacing o f RGO-1100˚C films was decreased to 3.51 Å, which is smaller than of RGO, suggesting that thermal annealing further removed the residual oxygen-con- taining functional group on the surface of RGO sheets. The XRD results clearly indicated tha t t he r mal annea l in g enables a better ordering of the 2D sheets. Note that d-spaci ng of t he RG O-1100˚C film is slightl y gre ater t han, but quite close to, that of graphene la yers in graph ite, indica ting that RGO -1100˚C sheets are similar to the pris- tine sheets. The slightly increased d-spaci ng of RGO -1100˚C films can be attributed to the presence of a small amount of residual oxygen-contai ning functiona l groups o r other str uctural d efects. Figure 5(a)-(c) shows the FT-IR spectra of GO, RGO and RGO-1100˚C films. The FT-IR spectrum of GO exhibits a strong and broad band at 3400 cm−1, whic h is due to the O-H stretching vibration. The band at 1724 cm−1 is related to the C=O stre tching motion s of COOH gro up s at the edge s of the s heets [ 36]. T he b a nd at 1 62 2 cm−1 can be due to the O-H bending vibration of absorbed water. The peak at 1384 cm−1 can be attrib uted to OH deformation vibration mode [36]. The spectrum also shows two peaks at 1233 and 1074 cm−1, being originated from the C-O stretching vibrations of epoxy and alkoxy, respectively [36] [37] . In the FT-IR spectrum of the RGO, the peaks at 1724, 1834 and 1233 cm−1 were disappeared. The intensity of peak at 1089 cm−1 (C-O), 1725 cm−1 (C=O) and broad peak at 3400 cm−1 were markedly reduced, indicating the removal of the hydroxyl and carboxylic acid groups. It did not completely disappear because of the mild reaction conditions. In addition, there are three new bands at 1557, 1433 and 1198 cm−1 appeared in the spectrum of RGO. The band at 1557 corresponds to aromatic C=C bonds [36], indicating the restor ation of the sp2 carbo n netwo rks duri ng chemical reduction. The two bands at 1433 and 1198 are attributed to sp3 C-N [38] and C-N [39] stretching modes, re- spectively. After thermal annealing, the spectrum of RGO-1100˚C shows only two bands at 1545 (C=C) and 1213 (C-N stretchi ng) cm−1, suggesting the effectiveness of thermal annealing in re moving the residual oxygen functional groups on the sur face of RGO sheets. Figure 4 . XRD patterns of raw graphite powders, GO, RGO and RGO-1100˚C films.
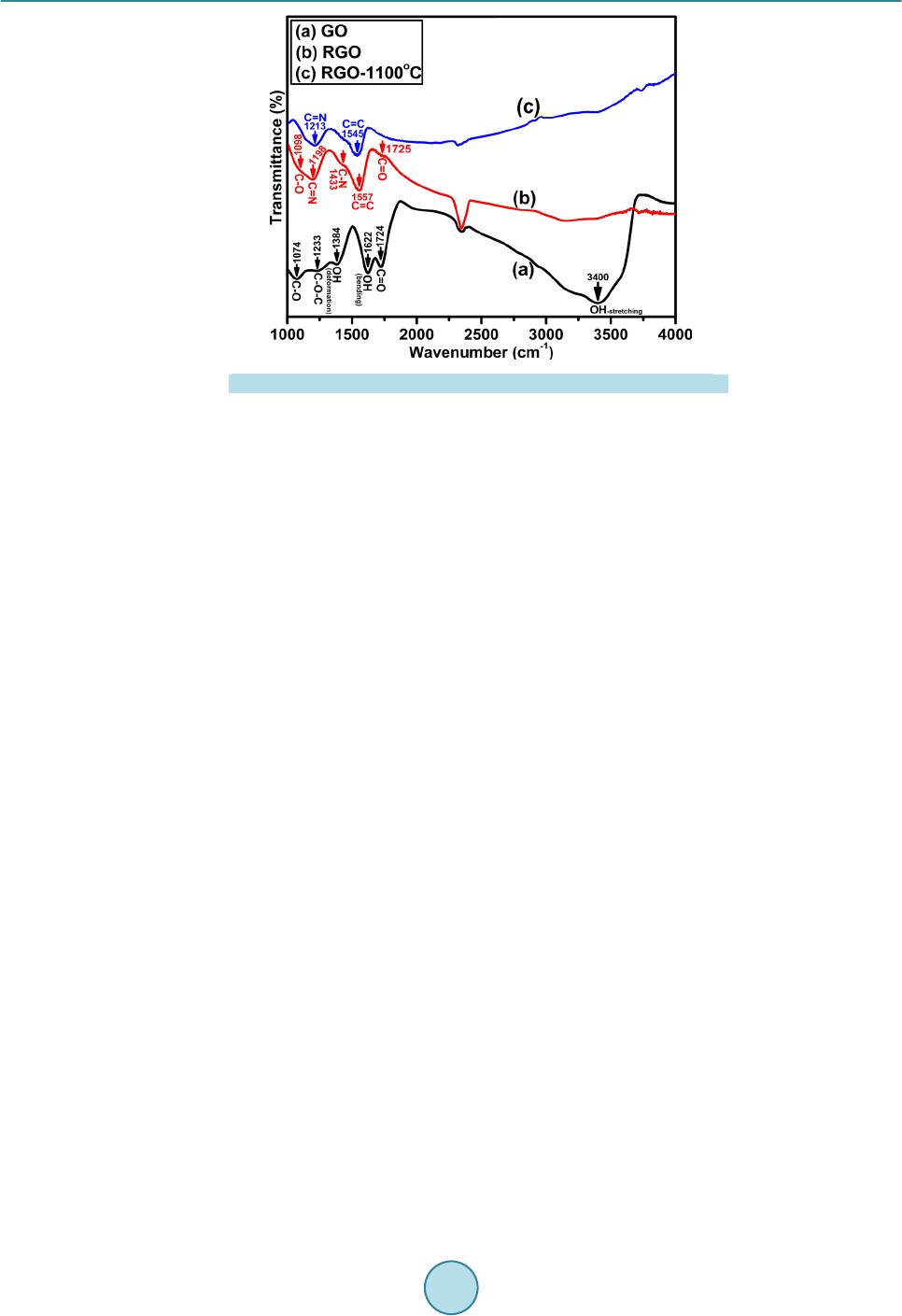 T. Van Khai et al. Figure 5. FT-IR spectra of (a) GO; (b) RGO; and (c) RGO-1100˚C films. Figure 6 shows Raman spectra of graphite powders, GO, RGO and RGO-1100˚C films. It clearly indicates that the graphite powder has the best crystal quality. Graphite has a prominent G band at 1582 cm−1 which i s as- signed to the fist-order scattering of the E2g mode observed for sp2 carbon domains and broad D band at 1358 cm−1 is caused by sp3-hybridized carbon, structural defects, carbon amorphous or edge planes that can break the symmetry and selection rule [40]. In the Raman spectrum of GO, the band G band is broadened and shifted to 1602 cm−1, while the D band at 1352 cm−1 becomes prominent, indicating the destruction of conjugated system in graphite. After GO was chemically reduced, the D band became narrower and more prominent whilst the G band shifted from 1602 cm−1 to 1596 cm−1, possibly due to increase of the number of sp2 car b on i n t he gr ap hene sheets. Moreover, after the subsequent thermal reduction at 1100˚C, the G band was further shifted to 1588 cm-1, suggesting that annealing removed the residual oxygen functional groups from the RGO sheets and more sp2 carbon networks were generated in the graphene sheet. The intens ity r atio of D band to G band (I D/IG) is usual l y used to measure degree of disordered carbon, as expressed by the sp3/sp2 carbon ratio [41] and an increase of ID/IG means the degradation of crystallinity of graphitic materials. The ID/IG ratios of graphite, GO, RGO and RGO-1100˚C was calculated to be 0.10, 0.85, 1.11 and 1.59, respectively. The increase of ID/IG can be attributed to a decrease in the average size but an increase in the number of sp2 do mai n up o n re d uction [42], b ut this effect obviously cannot be considered as the healing or repairing of defects in GO. XPS is a powerful tool to identify the elemental composition in bulk materials. Furthermore, by analysis of binding energy (BE) values, the feature of binding between carbon and oxygen is confirmed. Figure 7 shows the XPS survey spectra of graphite powders, GO, RGO and RGO-1100˚C films. Only car bon, ox ygen and nitr ogen species are detected, the atomic percentage (at%) of each element was calculated from the survey spectra, and the result was summarized in Table 1. In the survey scan XPS spectra, the peaks at around 285.5, 399.8 and 534.0 eV correspond to C1s, N1s and O1s core-level, respectively. The effective reduction is clear because the conte nt of ox ygen ato ms of RGO and RGO-1100˚C significantly reduces compared with GO. T he oxyge n con- tent decreased in the order of GO > RGO > RGO-1100˚C. High resolution XPS of the as-made samples were further performed to analyze the change of individual groups during the reduction process; the relative composition of individual groups was estimated by the percen- tage of the certain group (the area of peak divided by the total area of all peaks). Figure 8 shows t he hig h reso- lution C1s XPS spectra of graphite, GO, RGO and RGO-1100˚C films. For the graphite material, the C1s peak can be fitted to three components located at 284.5, 285.6 and 286.6 eV. The main peak at 284.5 eV corresponds to b inding ener gy of sp 2 graphitic bonds (C=C), i ndicating that most of the carb on atoms in the graphite are ar- range d i n a c onj u ga te d honeycomb lattice (89.9% area of C=C bonds). The other two peaks located at 285.6 and 286.6 eV are attributed to C-OH (hydroxyls) C-O-C (epoxy/ether) respectively. The C1s XPS spectrum of GO clearly indicates a considerable degree of oxidation with the content of oxygen element increased to 31.5 at% while the ar ea composition of C=C bonds decreased to 52.0%, which results from harsh oxidation and destruc- tion of the sp2 atomic structure graphite [43] [44]. There are six different kinds of carbon atoms, located
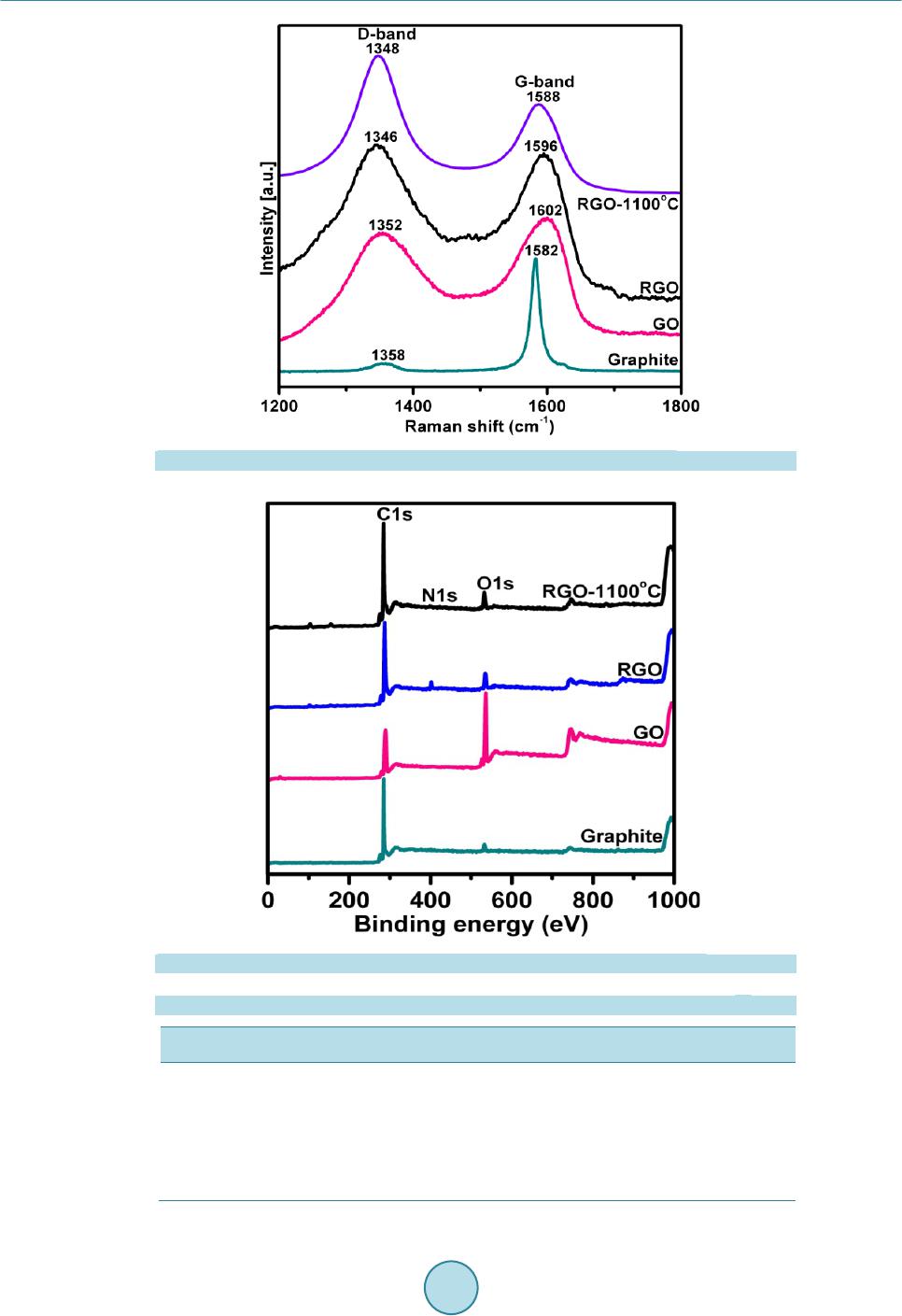 T. Van Khai et al. Figure 6. Raman sp ectra of raw graphite, GO, RGO and RGO-1100˚C films. Figure 7 . Wide -s can XPS spectra of graphi te, GO, RGO and RGO -1100˚C films. Table 1. Atomic concentration of C, N, and O of Graphite, GO, RGO and RGO-1100˚C. Sample C (%) N (%) O (%) Graphite 96.5 -- 3.5 GO-dr ied 68.5 -- 31.5 RGO 77.4 2.6 20.0 RGO-1100˚C 94.8 1.2 4.0
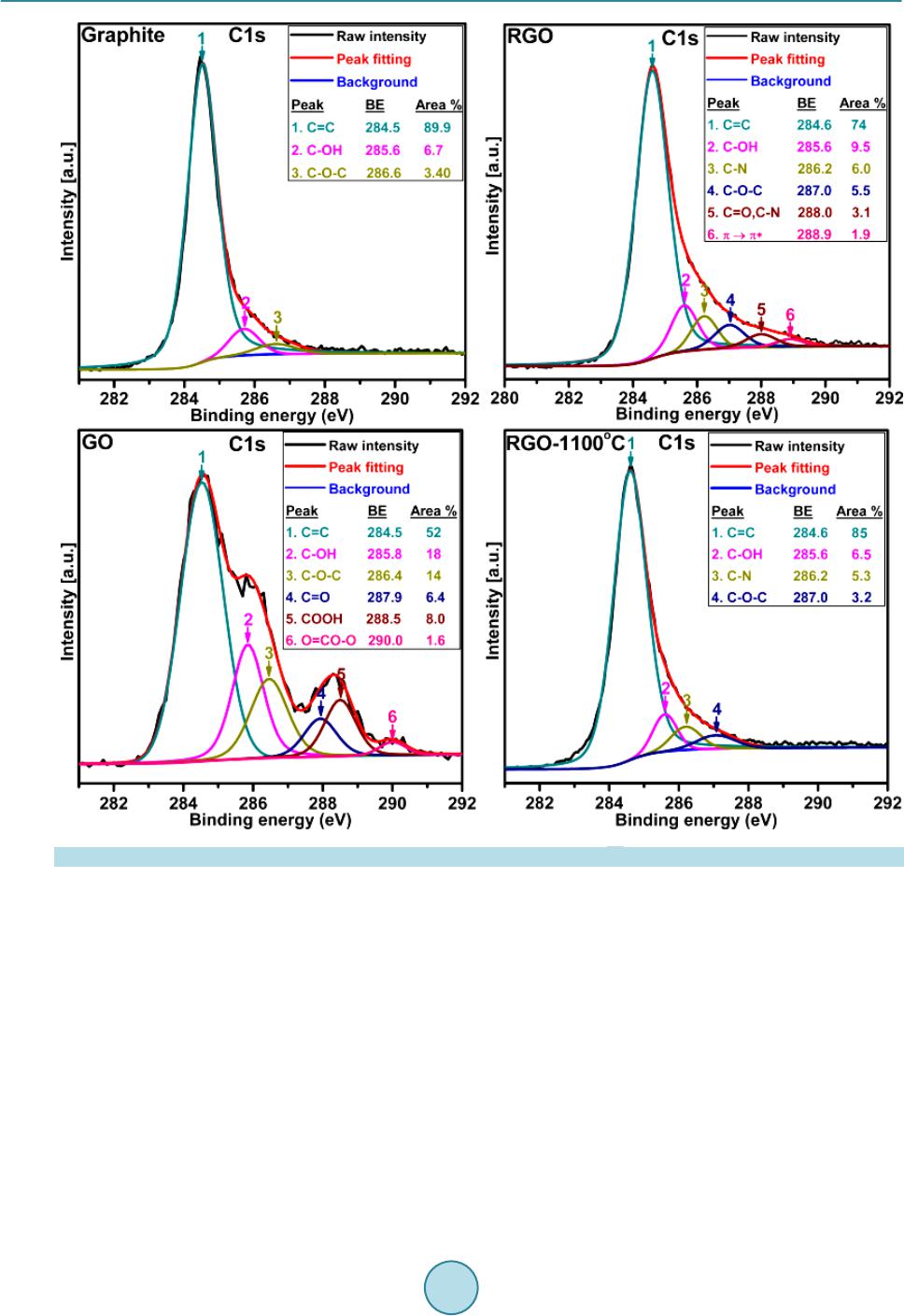 T. Van Khai et al. Figure 8 . High resolution C1 XPS spectra of Graphite, GO, RGO and RGO-1100˚C films. at 284.5, 285.8, 286.4, 287.9, 288.5 and 290.0 eV, correspondingly exist in different functional groups: C=C, C-OH, C-O-C, C=O, COOH (acid s) and O=CO-O (carbonates) groups, respectively. This indicated that the rich groups are contained within the GO. After chemical reduction of GO, the relative contribution of the compo- nents associ ated wit h oxyge n was found to decre ase very si gnificant , this i s in agr eement with p reviou s repor ts from the literature [9 ] [45]. I t is wort h noting t hat the cont ent of ox ygen eleme nt decre ased t o 20 at% while the area composition of C=C bonds increased to 70.0% in comparison to GO, indicating a significant recovery of sp2 carbo n network. In this case, the C1s peak can fitted to six compo nent s loc ate d at 28 4.6, 285. 6, 28 6.2, 287.0, 288.0 and 288.9 eV. The peaks located at 284.6, 285.6 and 287.0 eV correspond to energy of C=C, C-OH and C-O-C gro ups, resp ective ly. Conc urrently, two new peaks at 286.2 and 288.0 eV can be ascribed to two differ- ent C-N bonds, corresponding to the N-sp2C and N-sp3C bonds , respect ively, or iginatin g fr om the substitution of the N atoms, defects or the edge of the graphene sheets [46] [47]. During t he chemi cal re duction, oxygen f unc- tional gr oups i n the as-made GO were responsible for reactions with hydrazine monohydrate (NH4H2O) to form C-N bonds. Additionally, it is remarkable that the π → π* shakeup satellite peak around 288.9 eV, a characteris- tic of aromatic or conjugated systems, appeared after hydrazine reduction. Furthermore, after the thermal an-
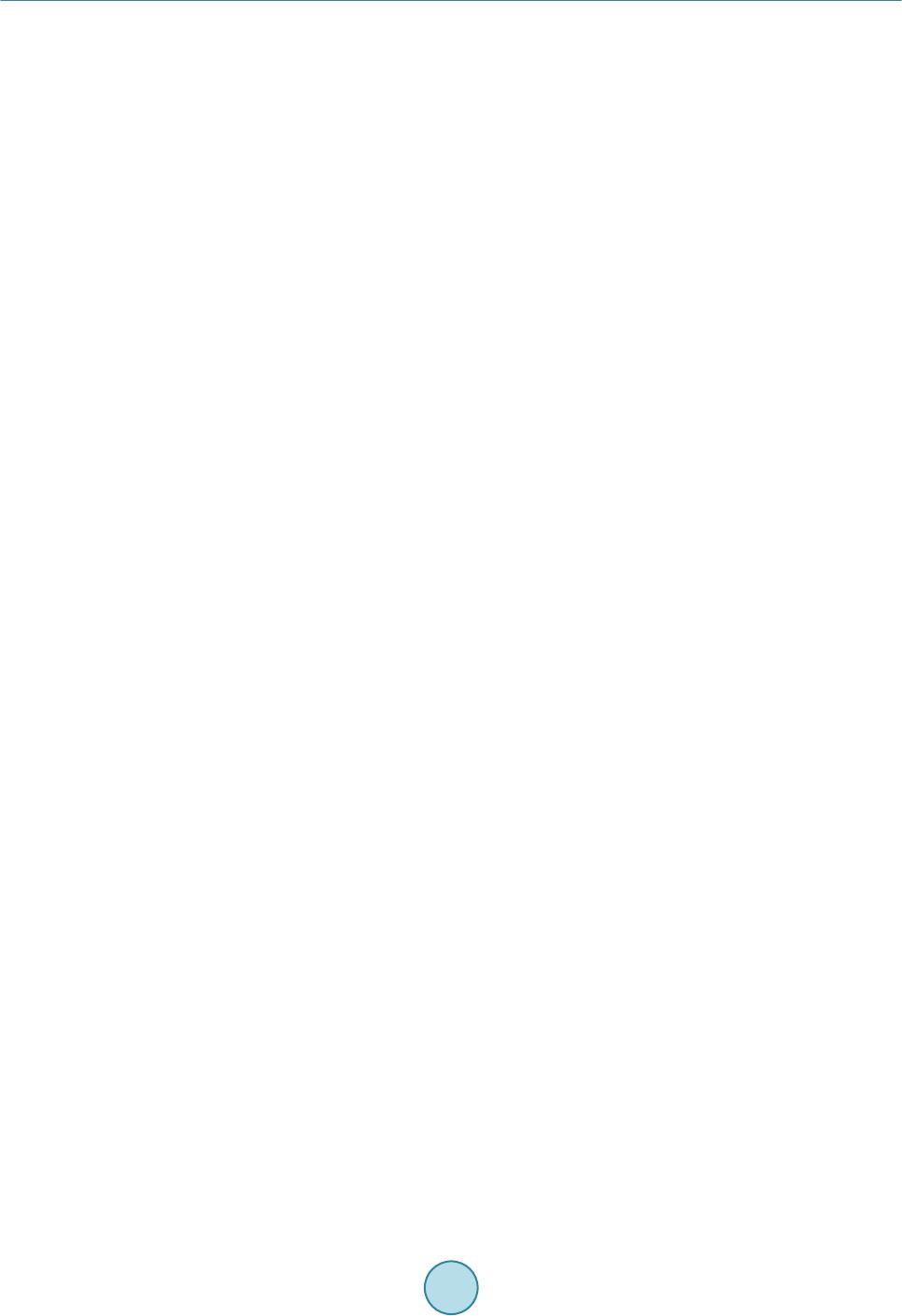 T. Van Khai et al. nealing, it is clearly found that the residual oxygen functional group (C-OH and C-O -C) was considerably re- duced and some of them even disappeared (C=O), meanwhile the composition of C=C bonds was increased up to 85.0%, clearly indicating the effeteness of thermal annealing in removing oxygen functional groups from GO and restoration of sp2 carbon networks. In addition, the intensity of the C-N peak was also reduced while the peak at 288.9 eV (π → π*) disappeared in the spectrum of RGO-1100˚C sample. 3.2. Electrical Property To study the electrical conductivity of the as-synthesized samples, we investigated the current-voltage (I-V) char acter istic us ing fo ur poi nt pr obe with Ke ithle y 2400 Source -meter. Figure 9 sho ws the I-V characteristic of GO, RGO and RGO-1100˚C samples. It is observed that all the samples exhibited linear I-V relation with the voltage in range of −1.0 to +1.0 V. The I-V slope of GO is close to zero. Before reduction, the GO behaved close-to-insulating material [48], which can be attributed to the high oxygen content in the form of functional groups contained by GO. It is widely known that the GO structure is predominantly amorphous due to distor- tions from high fraction of sp3-O. Moreover, due to the random distribution, the sp2-hybridized benzene rings are separated by sp3-hybridized rings, thus leading to the insulating GO [49]. However, the I-V slope of RGO significantly increased after chemical reduction, indicating that the electrical conductivity of RGO was consi- derably enhanced. The enhanced electrical conductivity of RGO can be due to chemical removal of oxygen functi o nal gr oup s a nd si multaneo us restor ation of sp2 c arb on ne t works d ur ing c he mical re duct ion. Ho weve r, the electrical co nductivity of RGO is still lo wer than that o f graphite [32]. It indicates that the structure of GO can- not be completely restored to graphitization by hydrazine monohydrate. To be suitable for practical applications, the conductivity of RGO needs to be improved. As a consequence of better ordering and additional deoxy-gena- tion by thermal annealing at 1100˚C in H2 ambient, the electrical conductively of RGO is found to further in- crease. The graphitization temperature of carbon materials is known to be 1100˚C [13], at which the residual oxyge n funct ional gr oups in RGO wil l be co mplet ely eli minated [50], contributing to the i mprovement o f elec- trical conductivi ty. From the linear I -V curve s the cond ucti vit y of GO , RGO a nd RGO -1100˚C was estimated to be 4.2 × 10−4, 24 and 210 S/cm, corresponding to GO, RGO and RGO-1100˚C, respectively, which is compara- ble to that pr e sented by Geng et al. [51]. The I-V measurement data, in conj uncti on with XPS and Raman res ults, reveal that the conductivity of GO increased with increasing sp2 carbon content and with increasing ID/IG. A similar result was reported by Lopez et al. [52], in which it was observed that the CVD-GO exhibited an ap- proximately linear rise of electrical conductively with increasing ID/IG. The improved electrical conductivity of GO by heating has been st udied intensively [53][54]. 3.3. Sensing Property One of the most important issues of gas sensor is reproducibility. Therefore, the RGO and RGO-1100˚C based gas sensor developed in this research was exposed to a repeated cycling of the ambient gas with different nitro- gen dioxide (NO2) concentrations. Figure 10 shows the normalized change of current in the RGO and RGO- 1100˚C samples as function of time for different concentration of NO2 ranging from 10 ppm increase to 50 ppm. NO2 appeared to act as a p-typed dopant [55] for both RGO and RGO-1100˚C films, resulting in a decrease in the resistance. The current of the RGO and RGO-1100˚C device increased with increasing flow of NO2. How- ever, it is obser ved that the sensitivi ty of RGO-1100˚C based device to NO2 is higher tha n that of R GO, pr oba- bly due to higher cond uctivity of RGO-1100˚C film. Similar results were fou nd in the previous studie s [56] [57]. The operational principle of graphene devices is based on changes in their electrical conductivity due to gas molecules adsorbed on graphene’s surface and acting as donors or acceptors, similar to other solid-state senso rs [3] [58]-[60]. It was further shown that the adsorbed molecules change the local carrier concentration in gra- phene one by one electron, which leads to step-like c hange s in resist ance . In ano ther st udy, it was obser ved tha t when graphene-gas sensor is exposed to NO2 gas molecules, the carrier concentration of graphene is changed whic h lead s to the c hange s in t he cond uctanc e, and c onse quent ly, in t he curr ent, t his p henomeno n is c onsid ered as sensing mechanism [61]. Based on this mechanism, Lu et al. [62] showed that the sensing behavior of the RGO was dependent on its electrical conductance. It was suggested that RGO was highly responsive to NO2 most likely due to the recovery of many graphitic carbon atoms as active sites for NO2 adsorption. Vacancies or small holes were probabl y created i n thermal treat ment a nd these defects may also serve as absorption sites for ga- seous molecules [62]. It was known that the higher electrical conductance in the RGO, the more clusters
 T. Van Khai et al. Figure 9 . Current-voltage ch ar acteristics of (a) GO; (b) RGO; and (c) RGO-1100˚C films. Figure 10. Reversibility and sensitivity of the (a) RGO and (b) RGO-1100˚C based sensor for detection of different concen- trations of NO2 at 200˚C in air. of gra p hit ic ca r bo n a toms ca n be r est or e d i n the gra p he ne , a nd t hus be tt er se nsing b e ha vior . B a sed o n thi s r es ult , it can be concluded that the RGO-1100˚C films have great p otential for ultra-sensiti ve gas senso r b y the op timi- zation of device structure. The detection of a single gas molecule can be achieved by using RGO-1100˚C based gas sensor since the change in the electrical conductivity of the graphene is quantized in response to NO2 mole- cule [19]. Moreover, the sensitivity can be further improved by modification of RGO by adding noble metals [63] or metal oxides [64] [65]. 4. Conclusion Highly conductive graphene films were fabricated by spray deposition of the as-prepared RGO dispersion on the quartz substrate, followed by thermal treatment at 1100˚C under H2 ambient. By analyzing XP S, Raman, XRD and FTIR spectra, it was found that the structure of GO was greatly changed upon reduction. Thermal treatment of RGO sheets resulted in further increasing sp2 carbon content, although decrease in the average size of sp2 domains (ID/IG increased). Therefore, the hi gher electrical conductivity of the RGO-1100˚C film compared with that of the RGO and GO films is likely attributed to the creation of more graphitic carbon atoms during the 1100˚C anneali ng. F urther, a tin y gas-sensor based on the RGO-1100˚C film exhibited great sensitivit y and fast response to NO2 gas. The simple, low-cost and efficient method for the production of large quantities of gra-
 T. Van Khai et al. phene films described here could be developed for a variety of advanced applications, such as environmental monitoring, sensing in chemi cal pro cessing pla nts, and gas de tecti on for counter-terrorism. Acknowledgements This work was supported by the National Foundation for Science and Technology Development of Viet Nam (NAFOSTED, code 104.03.2013.39). References [1] Zhu, Y., Murali, S., Cai, W., Li, X., Suk, J.W., Potts, J.R. and Ruoff, R.S. (2010) Graphene and Graphene Oxide: Syn- thesis, Properties, and Applications. Advanced Materials, 22, 3906-3924. http://dx.doi.org/10.1002/adma.201001068 [2] Rao, C.N.R., Sood, A.K., Subrahmanyam, K.S. and Govindaraj, A. (2009) Graphene: The New Two-Dimensional Nanomaterial. Angewandte Chemie International Edition, 48, 7752-7777. http://dx.doi.org/10.1002/anie.200901678 [3] Basnayaka, P.A., Ram, M.K., Stefanakos, L. and Kumar, A. (2013) Graphene/P olypyrrole Nano composite as E lectro- chemical S upercap acitor E lectrode: Elect r ochemical Impedance St udies. Graphene, 2, 81-87. http://dx.doi.org/10.4236/graphene.2013.22012 [4] Novoselov, K.S., Geim, A.K., Morozov, S.V., Jiang, D., Zhang, Y., Dubonos, S.V., Grigorieva, I.V. and Firsov, A.A. (2004) Electric Field Effect in Atomically Thin Carbon Films. Science, 306, 666-669. http://dx.doi.org/10.1126/science.1102896 [5] Hummer s, W.S. an d Offeman, R.E. (1958) Preparation of Graphit ic Oxide. Journal of the American Chemical Society, 80, 1339 . http://dx.doi.org/10.1021/ja01539a017 [6] Lerf, A., He, H., Forster, M. and Kliowski, J. (1998) Structure of Graphite Oxide Revisited. Journal of Physical Che- mistry B, 102, 4477-4482. http://dx.doi.org/10.1021/jp9731821 [7] He, H., Riedl, T., Lerf, A. and Klinowski, J. (1996) Sol id-Stat e NMR Studies of the Structure of Graphite Oxide. Jour- nal of Physical Chemistry, 100, 19954-19958. http://dx.doi.org/10.1021/jp961563t [8] Watcharotone, S., Dikin, D.A., Stankovich, S., Piner, R., Jung, I., Dommett, G.H.B., Evmenenko, G., Wu, S.E., Chen, S.F., Liu, C.P., Nguyen, S.B.T. and Ruoff, R.S. (2007) Graphene-Silica Composite Thin Films as Transparent Con- ductors. Nano Let ters, 7, 1888-1892. http://dx.doi.org/10.1021/nl070477+ [9] Eda, G., Fanchini, G. and Chhowalla, M. (2008) La rge-Area Ultrathin Films of Reduced Graphene Oxide as a Trans- parent and Flexible Electro nic Material. Nature Nanotechnology, 3, 270-274. http://dx.doi.org/10.1038/nnano.2008.83 [10] Gilje, S., Han, S., Wang, M., Wang, K.L. and Kaner, R.B. (2007) A Chemical Route to Graphene for Device Applica- tions. Nano Let ters, 7, 3394 -3398. http://dx.doi.org/10.1021/nl0717715 [11] Li, D., Müller, M.B., Gilje, S., Kaner, R.B. and Wallace, G.G. (2008) Processable Aqueous Dispersions of Graphene Nanosheets. Nature Nanotechnology, 3, 101-105. http://dx.doi.org/10.1038/nnano.2007.451 [12] Wang, X., Zhi, L.J. and Muellen, K. (2008) Transparent, Conductive Graphene Electrodes for Dye-Sensitized Solar Cells. Nano Letters, 8, 323-327. http://dx.doi.org/10.1021/nl072838r [13] Becerril, H.A., Mao, J., Liu, Z.F., Stoltenberg, R.M., Bao, Z.N. and Chen, Y.S. (2008) Evaluation of Solution-Proc- essed Red uced Graphene Oxide Films as Transparent Conductors. ACS Nano, 2, 463-470. http://dx.doi.org/10.1021/nn700375n [14] Novoselov, K.S., Geim, A.K., Morozov, S.V., Jiang, D., Katsnelson, M.I., Grigorieva, I.V., Dubonos, S.V. and Firsov, A.A. (2005) Two-Dimensional Gas of Massless Dir ac Fermions in Graphene. Nature, 438, 197-200. http://dx.doi.org/10.1038/nature04233 [15] Berger, C., Song, Z.M., Li, X.B., Wu, X.S., Brown, N., Naud, C., Mayo, D., Li, T.B., Hass, J., Marchenkov, A.N., Conrad, E.H., First, P.N. and de Heer, W.A. (2006) Electronic Confinement and Coherence in Patterned Epitaxial Graphene. Scien ce, 312, 1191-1196. http://dx.doi.org/10.1126/science.1125925 [16] Novoselov, K.S., McCann, E., Morozov, S.V., Fal’ko, V.I., Katsnelson, M.I., Zeitler, U., Jiang, D., Schedin, F. and Geim, A.K. (2006) Unconventional Quant um Hall E ffect and Berr y’s P h ase of 2π in Bilayer Grap h ene. Na ture Ph ysics, 2, 177-180. http://dx.doi.org/10.1038/nphys245 [17] Zhang, Y.B., Small, J.P., Amori, M.E.S. and Kim, P. (2005) Electric Field Mo dulation of Galvano magnetic P roperties of Mesoscopic Graphite. Physical Review Letters, 94, Article ID: 176803. http://dx.doi.org/10.1103/PhysRevLett.94.176803 [18] Lemme, M.C., Echtermeyer, T.J., Baus, M. and Kurz, H. (2007) A Graphene Fiel d-Effect Device. IEEE Electron De- vice Letters , 28, 282-284. http://dx.doi.org/10.1109/LED.2007.891668 [19] Schedi n, F., Geim, A.K., Morozov, S.V., Hill, E.W., Blake, P., Katsnelson, M.I. and Novoselov, K.S. (2007) Detection
 T. Van Khai et al. of Individual Gas Molecules Adsorbed on Graphene. Nature Materials, 6, 652-655. http://dx.doi.org/10.1038/nmat1967 [20] Sundaram, R.S., Navarro, C.G., Balasubramanian, K., Burghard, M. and Kern, K. (2008) Electrochemical Modification of Graphene. Advanced Materials, 20, 3050-3053. http://dx.doi.org/10.1002/adma.200800198 [21] Ang, P.K., Chen, W., Wee, A.T.S. and Loh, K.P. (2008) Solution-Gated Epit axial Graphene as pH Sensor. Journal of the American Chemi cal Society, 130, 14392-14393. http://dx.doi.org/10.1021/ja805090z [22] Leenaerts, O., Partoens, B. and Peeters, F.M. (2008) Adsorption of H2O, NH3, CO, NO2, and NO on Graphene: A First-Principles Study. Physical Review B, 77, Article ID: 125416. http://dx.doi.org/10.1103/PhysRevB.77.125416 [23] Huang, B., Li, Z.Y., Liu, Z.R., Zhou, G., Hao, S.G., Wu, J., Gu, B.L. and Duan, W.H. (2008) Adsorption of Gas Molecules on Graphene Nanoribbons and Its Implication for Nanoscale Molecule Sensor. The Journal of Physical Chemistry C, 112, 13442-13446. http://dx.doi.org/10.1021/jp8021024 [24] Peng, S. and Cho, K. (2003) Ab Initio Study of Doped Carbon Nanotube Sensors. Nano Letters, 3, 513-517. http://dx.doi.org/10.1021/nl034064u [25] Yeung, C.S., Liu, L.V. and Wang, Y.A. (2008) Adsorption of Small Gas Molecules onto Pt-Doped Single-Walled Carbon Nanotubes. The Journal of P hysical Chem istry C, 112, 7401-7411. http://dx.doi.org/10.1021/jp0753981 [26] Ao, Z.M., Yang, J., Li, S. and Jiang, Q. (2008) Enhancement of CO detection in Al Doped Graphene. Chemical Phys- ics Letter s , 461, 276-279. http://dx.doi.org/10.1016/j.cplett.2008.07.039 [27] Qazi, M., Vogt, T. and Koley, G. (2007) Trace Gas Detect ion Using Nan ostructur ed Graphite Layers. A pplied Physics Letters, 91, Article ID: 233101. http://dx.doi.org/10.1063/1.2820387 [28] Jung, I., Dikin, D., P ar k, S., Cai, W., Mielke, S.L. and Ruoff, R.S. (20 08) Effect of Water V apor on Electri cal Proper- ties of In dividual R educed Graphene Oxide S heets. The Journal of Physical Chemistry C, 112, 20264-20268. http://dx.doi.org/10.1021/jp807525d [29] Robinson, J.T., Perkins, F.K., Snow, E.S., Wei, Z. and Sheehan, P.E. (2008) Reduced Graphen e Oxide Molecu lar Sen- sors. Nano Letters, 8, 3137-3140. http://dx.doi.org/10.1021/nl8013007 [30] Fowler, J.D., Allen, M.J., Tung, V.C., Yang, Y., Kaner, R.B. and Weiller, B.H. (2009) Practical Chemical Sensors from Chemically Derived Graph ene. AC S Nano , 3, 301-306. http://dx.doi.org/10.1021/nn800593m [31] Wu, Z.S., Ren, W., Gao, L., Liu, B., Jiang, C. and Cheng, H.M. (2009) Synthesis of High-Quality Graphene with a Pre-Determin ed Number o f Layers. Carbon, 47, 493-499. http://dx.doi.org/10.1016/j.carbon.2008.10.031 [32] Stankovich, S., Dikin, D.A., Piner, R.D., Kohlhaas, K.A, Kleinhammes, A., Jia, Y., Wu, Y., Nguyen, S.B.T. and Ruoff, R.S. (2007) Synthesis of Graphene-Based Nano sheets via Chemical Redu ction of Exfoliated Graph ite Oxide. Carbon, 45, 1558-1565. http://dx.doi.org/10.1016/j.carbon.2007.02.034 [33] Th ema, F.T., Molo to, M.J., Dikio, E.D., Nyangiwe, N.N., Ko tsedi , L., Maaza, M. and Khenfouch, M. (2013) Synthesis and Characterization of Graphene Thin Films by Chemical Reduction of Exfoliated and Intercalated Graphite Oxide. Journal of Chemistry, 2013, Article ID: 150536. http://dx.doi.org/10.1155/2013/150536 [34] Muthoosamy, K., et al. (2015) Exceedingly Biocompatible and Thin-Layered Reduced Graphene Oxide Nanosheets Using an Eco-Friendly Mushroom Extract Strategy. International Journal of Nanomedicine, 10, 1505-1519. [35] Hung, P.V., Cuong, T.V., Hur, S.H., Shin, E.W., Kim, J.S., Chung, J.S. and Kim, E.J. (2010) Fast and Simple Fab rica- tion o f a Large Transparen t Chemically-Converted Graphene Film by Spray-Coating. Carbon, 48, 1945-1951. http://dx.doi.org/10.1016/j.carbon.2010.01.062 [36] Hontorialucas, C., Lopezpeinado, A.J., Lopezgonzalez, J.D.D., Rojascervantes, M.L. and Martinarand a, R.M. (1995) Study of Oxygen-Containing Groups in a Series of Graphite Oxides: Physical and Chemical Characterization. Carbon, 33, 1585 -1592. http://dx.doi.org/10.1016/0008-6223(95)00120-3 [37] Chandra, V. and Kim, K.S. (2011) Highly Selective Adsorption of Hg2+ by a Polypyrrole-Reduced Graphene Oxide Composite. Chemical Communications, 47, 3942-3944. http://dx.doi.org/10.1039/c1cc00005e [38] Ricci, M., Trinquecoste, M., Auguste, F., Canet, R., Delhaes, P., Guimon, C., Pfister-Guillouzo, G., Nysten, B. and Issi, J.P. (1993) Relationship between the Structural Organization and the Physical Properties of PECVD Nitrogenated Carbons. Journal of Materials Research, 8, 480-488. http://dx.doi.org/10.1557/JMR.1993.0480 [39] Ren, Z.M ., Lu, Y.F., Song, W.D., Chan, D.S.H., Low, T.S., Gamani, K., Chen, G. and Li, K. (1998) Studies of Carbon Nitride Thin Films Synthesized by KrF Excimer Ablation of Graphite in Nitrogen Atmosphere. MRS Proceedings, 526, 343-348. http://dx.doi.org/10.1557/PROC-526-343 [40] Ferrari, A.C., Meyer, J.C., Scardaci, V., Casiraghi, C., Lazzeri, M., Mauri, F., Piscanec, S., Jiang, D., Novoselov, K.S. and Roth, S. (2006) Raman Spectrum of Graphene and Graphene Layers. Physical Review Letters, 97, Article ID: 187401. http://dx.doi.org/10.1103/PhysRevLett.97.187401 [41] Ferrari, A.C. and Robertson, J. (2000) Interpretation of Raman Spectra of Disordered and Amorphous Carbon. Physi-
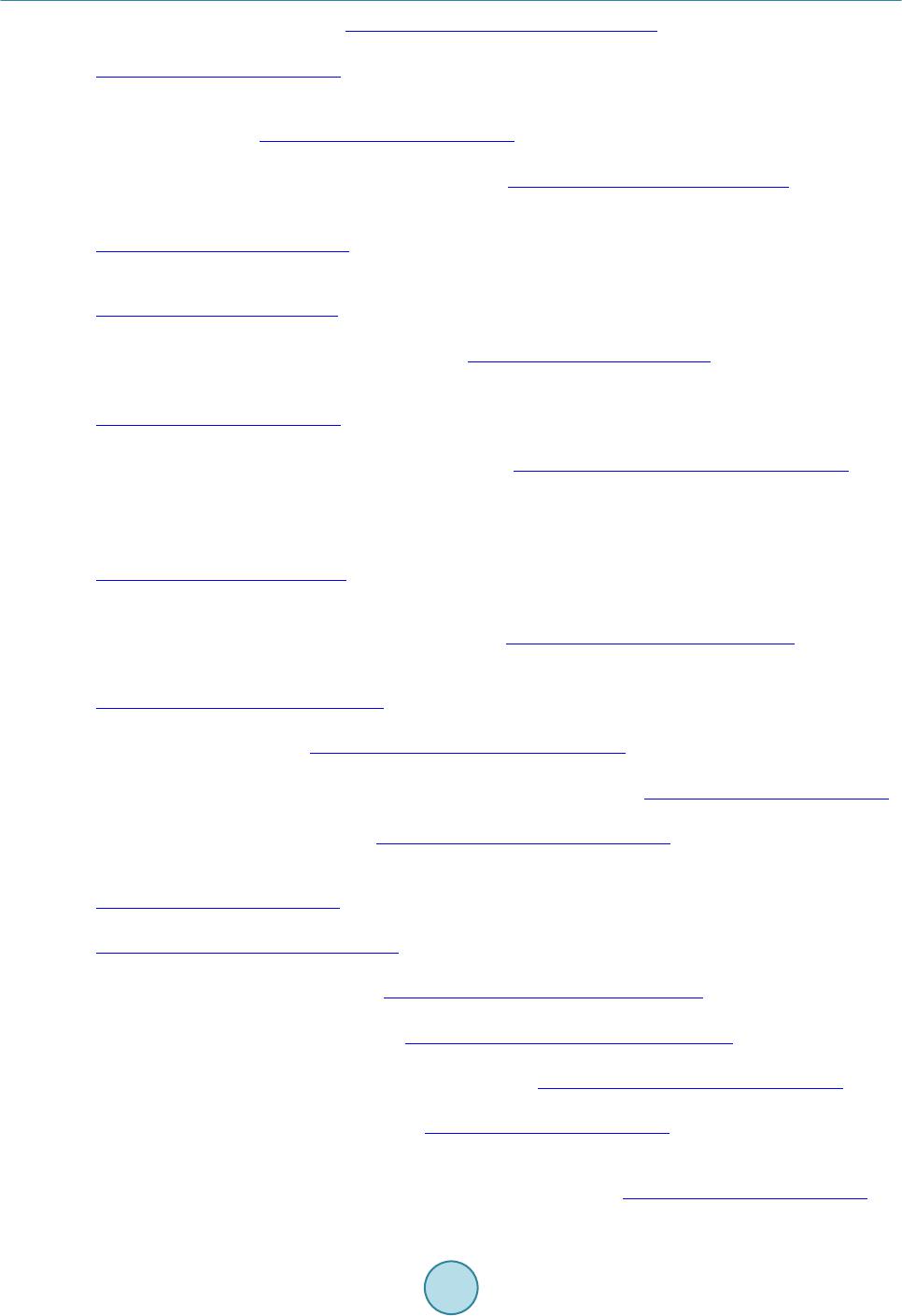 T. Van Khai et al. cal Revi ew B, 61, Article ID: 14095. http://dx.doi.org/10.1103/PhysRevB.61.14095 [42] Tuinstra, F. and Koenig, J.L. (1970) Raman Spectrum of Graphit e. The Journal of Chemical Physics, 53, 1126-1130. http://dx.doi.org/10.1063/1.1674108 [43] Jiang, B.J., Tian, C.G., Wang, L., Xu, Y.X., Wang, R.H., Qiao, Y.J., Ma, Y.G. and Fu, H.G. (2010) Facile Fabri cation of High Quality Graphene from Expandable Graphite: Simultaneous Exfoliation and Reduction. Chemical Communi- cations, 46, 4920-4922. http://dx.doi.org/10.1039/c0cc00383b [44] Chouciar, M., Thordarson, P. and Stride, J.A. (2009) Gram-Scale Production of Graphene Based on Solvothermal Synthesis and Sonication. Natur e N ano t e c hn ol o gy , 4, 30-33. http://dx.doi.org/10.1038/nnano.2008.365 [45] Park, S., An, J., Piner, R.D., Jung, I., Yang, D., Velamakanni, A., Nguyen, S.B.T and Ruoff, R.S. (2008) Aqueous Suspension and Characterization of Chemically Modified Graphene Sheets. Chemistry of Materials, 20, 65 92 -6594. http://dx.doi.org/10.1021/cm801932u [46] Wei, D.C., Liu, Y.Q., Yang, Y., Zhang, H.L., Huang, L.P. and Yu, G. (2009) Synthesis of N-Doped Graphene by Chemical Vapo r Dep osition an d Its E lectrical Properties. Nano Le tters, 9, 1752-1758. http://dx.doi.org/10.1021/nl803279t [47] Lee, D.H., Lee, W.J. and Kim, S.O. (2009) Highly Efficient Vertical Growth of Wall-Number-Selected, N-Doped Carbon Nanotube Arrays. Nano Letters, 9, 1427-1432. http://dx.doi.org/10.1021/nl803262s [48] Gómez-Navarro, C., Weitz, R.T., Bittner, A.M., Scolari, M., Mews, A., Burghard, M. and Kern, K. (2007) Electronic Transport Properties of Individual Chemically Reduced Graph ene Oxide Sheets. Nano Letters, 11, 3499-3503. http://dx.doi.org/10.1021/nl072090c [49] Ren, P.G., Yan, D.X., Ji, X., Chen, T. and Li, Z.M. (2011) Temperat ure Dependen ce of Graphene Oxide Reduced by Hydrazine Hydrate. Nanotechnology, 22, Article ID: 055705. http://dx.doi.org/10.1088/0957-4484/22/5/055705 [50] Kinoshita, K. (1998) Carbon: Electrochemical and Physicochemical Properties. John Wiley & Sons, New York, 560. [51] Geng, J., Liu, L., Yang, S.B., Youn, S.C., Kim, D.W., Lee, J.S., Choi, J.K. and Jung, H.T. (2010) A Simple Approach for Preparing Transparent Conductive Graphene Films Using the Controlled Chemical Reduction of Exfoliated Gra- phene Oxide in an Aqueous Suspension. The Journal of Physical Chemistry C, 114, 14433-14440. http://dx.doi.org/10.1021/jp105029m [52] Lopez, V ., Sundaram, R.S ., Gomez-Navarro, C., Olea, D., Burghard, M., Gomez-Herrero, J., Zamora, F. and Kern, K. (2009) Graphene Monolayers: Chemical Vapor Deposition Repair of Graphene Oxide: A Route to Highly-Conductive Graphene Monolayers . Advance d Materials, 21, 4683-4686. http://dx.doi.org/10.1002/adma.200990172 [53] Jin, M., Kim, T.H., Lim, S.C., Duong, D.L., Shin, H.J., Jo, Y.W., Jeong, H.K., Chang, J., Xie, S. and Lee, Y.H. (2011) Facile Ph ysical Route to Highly Crystalline Graphene. Adv anc e d Funct i onal Mate r ials, 21, 3496-3501. http://dx.doi.org/10.1002/adfm.201101037 [54] Wang, S.J., Geng, Y., Zheng, Q. and Kim, J.K. (2010) Fabrication of Highly Conducting and Transparent Graphene Films. Carbon, 48, 1815-1823. http://dx.doi.org/10.1016/j.carbon.2010.01.027 [55] Moser, J., Verdaguer, A., Jiménez, D., Barreiro, A. and Bachtold, A. (2008) The Environment of Graphene Probed by Electrostatic F orce Microscopy. Applied Physics Letters, 92, Article ID: 123507. http://dx.doi.org/10.1063/1.2898501 [56] Ko, G., Kim, H.W., Ahn, J., Park, Y.M., Lee, K.Y. and Kim, J. (2010) Graphene-Based Nitrogen Dioxide Gas Sensors. Curren t Applied P hysics, 10, 1002 -1004. http://dx.doi.org/10.1016/j.cap.2009.12.024 [57] Yavari, F., Chen, Z., Thomas, A.V., Ren, W., Cheng, H.M. and Koratkar, N. (2011) High Sensitivity Gas Detection Using a Macr oscop ic Three-Dimensional Graphene Foam Network. Scie ntific Report , 1, Article No.: 166. http://dx.doi.org/10.1038/srep00166 [58] Moseley, P.T. (1997) Solid State Gas Sensors. Measurement Science and Technology, 8, 223-237. http://dx.doi.org/10.1088/0957-0233/8/3/003 [59] Kong, J., Franklin, N.R., Zhou, C., Chapline, M.G., Peng, S., Cho, K. and Dai, H. (2000) Nanotube Molecular Wires as Chemical Sensor s. Science, 287, 622-625. http://dx.doi.org/10.1126/science.287.5453.622 [60] Collins, P.G., Bradley, K., Ishigami, M. and Zettl, A. (2000) Extreme Oxygen Sensitivity of Electronic Properties of Carbon Nanotubes. Science, 287, 1801-1804. http://dx.doi.org/10.1126/science.287.5459.1801 [61] Khaledian, M., Ismail, R., Saeidmanesh, M., Ghadiry, M. and Akbari, E. (2015) Sensitivity Modelling of Graphene Nanoscr oll Based NO2 Gas Sensors. Plasmonics, 10, 103 3-1040. http://dx.doi.org/10.1007/s11468-015-9905-6 [62] Lu, G., Ocola, L.E. and Chen, J.H. (2009) Gas Detection Using Low-Temperature Reduced Graphene Oxide Sheets. Applied Physics Letters, 94, Article ID: 083111. http://dx.doi.org/10.1063/1.3086896 [63] Penza, M., Gassano, G., Rossi, R., Alvisi, M., Rizzo, A., Signore, M.A., Dikonimos, T., Serra, E. and Giorgi, R. (2007) Enhancement of Sensitivity in Gas Chemiresistors Based on Carbon Nanotube Surface Functionalized with Noble Metal (Au, Pt) Nan oclusters. Applied Physics Lett er s , 90, Article ID: 173123. http://dx.doi.org/10.1063/1.2722207
 T. Van Khai et al. [64] Zhang, H., Feng, J.C., Fei, T., Liu, S. and Zhang, T. (2014) SnO2 Nanopart icles-Reduced Graphene Oxide Nanocom- posites for NO2 Sensing at Low Operatin g Temperature. Sensors and Actuators B: Chemical, 190, 472-478. http://dx.doi.org/10.1016/j.snb.2013.08.067 [65] Qin, J.W. , Cao, M.H., Li, N. and Hu, C.W. (2011) Graphene-Wrapped WO3 Nanoparticles with Improved Perfor- mances in Electrical Conductivity and Gas Sensin g Properti es. Journal of Materials Chemistry, 21, 17167-17171. http://dx.doi.org/10.1039/c1jm12692j
|