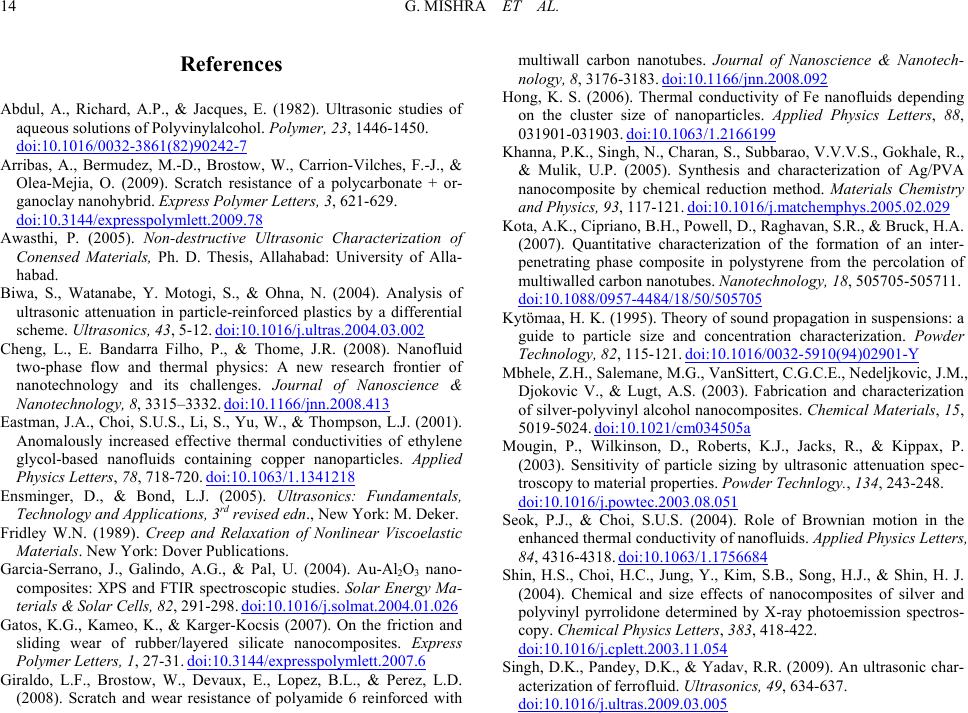
G. MISHRA ET AL.
14
References
Abdul, A., Richard, A.P., & Jacques, E. (1982). Ultrasonic studies of
aqueous solutions of Polyvinylalcohol. Polymer, 23, 1446-1450.
doi:10.1016/0032-3861(82)90242-7
Arribas, A., Bermudez, M.-D., Brostow, W., Carrion-Vilches, F.-J., &
Olea-Mejia, O. (2009). Scratch resistance of a polycarbonate + or-
ganoclay nanohybrid. Express Polymer Letters, 3, 621-629.
doi:10.3144/expresspolymlett.2009.78
Awasthi, P. (2005). Non-destructive Ultrasonic Characterization of
Conensed Materials, Ph. D. Thesis, Allahabad: University of Alla-
habad.
Biwa, S., Watanabe, Y. Motogi, S., & Ohna, N. (2004). Analysis of
ultrasonic attenuation in particle-reinforced plastics by a differential
scheme. Ultrasonics, 43, 5-12. doi:10.1016/j.ultras.2004.03.002
Cheng, L., E. Bandarra Filho, P., & Thome, J.R. (2008). Nanofluid
two-phase flow and thermal physics: A new research frontier of
nanotechnology and its challenges. Journal of Nanoscience &
Nanotechnology, 8, 3315–3332. doi:10.1166/jnn.2008.413
Eastman, J.A., Choi, S.U.S., Li, S., Yu, W., & Thompson, L.J. (2001).
Anomalously increased effective thermal conductivities of ethylene
glycol-based nanofluids containing copper nanoparticles. Applied
Physics Letters, 78, 718-720. doi:10.1063/1.1341218
Ensminger, D., & Bond, L.J. (2005). Ultrasonics: Fundamentals,
Technology and Applications, 3rd revised edn., New York: M. Deker.
Fridley W.N. (1989). Creep and Relaxation of Nonlinear Viscoelastic
Materials. New York: Dover Publications.
Garcia-Serrano, J., Galindo, A.G., & Pal, U. (2004). Au-Al2O3 nano-
composites: XPS and FTIR spectroscopic studies. Solar Energy Ma-
terials & Solar Cells, 82, 291-298. doi:10.1016/j.solmat.2004.01.026
Gatos, K.G., Kameo, K., & Karger-Kocsis (2007). On the friction and
sliding wear of rubber/layered silicate nanocomposites. Express
Polymer Letters, 1, 27-31. doi:10.3144/expresspolymlett.2007.6
Giraldo, L.F., Brostow, W., Devaux, E., Lopez, B.L., & Perez, L.D.
(2008). Scratch and wear resistance of polyamide 6 reinforced with
multiwall carbon nanotubes. Journal of Nanoscience & Nanotech-
nology, 8, 3176-3183. doi:10.1166/jnn.2008.092
Hong, K. S. (2006). Thermal conductivity of Fe nanofluids depending
on the cluster size of nanoparticles. Applied Physics Letters, 88,
031901-031903. doi:10.1063/1.2166199
Khanna, P.K., Singh, N., Charan, S., Subbarao, V.V.V.S., Gokhale, R.,
& Mulik, U.P. (2005). Synthesis and characterization of Ag/PVA
nanocomposite by chemical reduction method. Materials Chemistry
and Physics, 93, 117-121. doi:10.1016/j.matchemphys.2005.02.029
Kota, A.K., Cipriano, B.H., Powell, D., Raghavan, S.R., & Bruck, H.A.
(2007). Quantitative characterization of the formation of an inter-
penetrating phase composite in polystyrene from the percolation of
multiwalled carbon nanotubes. Nanotechnology, 18, 505705-505711.
doi:10.1088/0957-4484/18/50/505705
Kytömaa, H. K. (1995). Theory of sound propagation in suspensions: a
guide to particle size and concentration characterization. Powder
Technology, 82, 115-121. doi:10.1016/0032-5910(94)02901-Y
Mbhele, Z.H., Salemane, M.G., VanSittert, C.G.C.E., Nedeljkovic, J.M.,
Djokovic V., & Lugt, A.S. (2003). Fabrication and characterization
of silver-polyvinyl alcohol nanocomposites. Chemical Materials, 15,
5019-5024. doi:10.1021/cm034505a
Mougin, P., Wilkinson, D., Roberts, K.J., Jacks, R., & Kippax, P.
(2003). Sensitivity of particle sizing by ultrasonic attenuation spec-
troscopy to material properties. Powder Technlogy., 134, 243-248.
doi:10.1016/j.powtec.2003.08.051
Seok, P.J., & Choi, S.U.S. (2004). Role of Brownian motion in the
enhanced thermal conductivity of nanofluids. Applied Physics Letters,
84, 4316-4318. doi:10.1063/1.1756684
Shin, H.S., Choi, H.C., Jung, Y., Kim, S.B., Song, H.J., & Shin, H. J.
(2004). Chemical and size effects of nanocomposites of silver and
polyvinyl pyrrolidone determined by X-ray photoemission spectros-
copy. Chemical Physics Letters, 383, 418-422.
doi:10.1016/j.cplett.2003.11.054
Singh, D.K., Pandey, D.K., & Yadav, R.R. (2009). An ultrasonic char-
acterization of ferrofluid. Ultrasonics, 49, 634-637.
doi:10.1016/j.ultras.2009.03.005