 Vol.3, No.6, 488-495 (2011) Natural Science http://dx.doi.org/10.4236/ns.2011.36068 Copyright © 2011 SciRes. OPEN ACCESS Thermal response and optical absorptance of metals under femtosecond laser irradiation Anatoliy Y. Vorobyev, Chunlei Guo The Institute of Optics, University of Rochester, Rochester, USA; vorobyev@optics.rochester.edu, guo@optics.rochester.edu Received 15 March 2011; revised 10 April 2011; accepted 27 April 2011. ABSTRACT A detailed study on correlation between residual thermal response of a sample and its optical absorptance change due to laser-induced sur- face structural modifications in multi-shot fem- tosecond laser irradiation is performed. Ex- periments reveal an overall enhancement for residual thermal coupling and absorptance in air. Surprisingly, residual thermal coupling in air shows a non-monotonic dependence on pulse number and reaches a minimum value after a certain number of pulses, while these behaviors are not seen in absorptance. In vacuum, how- ever, both suppression and enhancement are seen in residual energy coupling although ab- sorptance is always enhanced. From these ob- servations, it appears that air plasma plays a dominant role in thermal coupling at a relatively low number of applied pulses, while the forma- tion of craters plays a dominant role at a high number of pulses. Keywords: Femtosecond Laser; Ablation; Residual Energy; Absorptance; Surface Structures 1. INTRODUCTION Many applications of femtosecond lasers, such as high-precision materials machining [1,2], nanotechnol- ogy [3-7], modification of optical properties of materials [8-12], thin film deposition [13], modification of wetting properties of solids [14-17], producing diamond-like materials [18], laser plasma thrusters [19], and biomedi- cine [17,20], are based on laser ablation of solids. Al- though femtosecond laser ablation have been extensively studied in the past [21-27], many of the physical mecha- nisms remain unclear. For example, recent studies have shown that the residual thermal energy in an irradiated metal sample abruptly increases following both sin- gle-pulse and pulse-train femtosecond laser ablation in a gas medium when the laser fluence is above a certain threshold [28,29]. In the case of pulse-train ablation [29], it was found that one contributing factor to the enhanced thermal coupling following a large number of applied pulses is an increase of sample absorptance due to sur- face structural modifications. However, we have shown that the surface modifications alone cannot fully explain the enhanced thermal energy deposition [29]. To further study the effects of the enhanced thermal coupling to metals, in this paper, we perform a shot-to-shot detailed study on how residual energy correlates to the absorp- tance change due to surface structural modifications. We observe an overall enhancement for residual thermal coupling and absorptance in air. Surprisingly, residual thermal coupling in air shows a non-monotonic depend- ence on pulse number and reaches a minimum value after a certain number of pulses, while these behaviors are not seen in absorptance. In vacuum, however, both sup- pression and enhancement are seen in residual energy coupling although absorptance is always enhanced. To explain these observations, we suggest that air plasma plays a dominant role in thermal coupling at a relatively low number of applied pulses while the formation of cra- ters plays a dominant role at a high number of pulses. 2. EXPERIMENTAL SETUP The laser used in the experiment is an amplified Ti:sapphire system generating 65-fs pulses of about 1.5 mJ/pulse at a 1-kHz repetition rate with the central wa- velength at 800 nm. Our experimental setup is shown in Figure 1. To produce ablation, the laser beam is focused onto a platinum (Pt) bulk sample at normal incidence. The Pt sample is mechanically polished before experi- ments. The number of laser pulses, N, delivered to the sample is controlled by a fast electromechanical shutter. Following pulse irradiation, some directly absorbed laser energy will be carried away by the ablated material, while a fraction of the absorbed pulse energy will remain in the surface layer of the sample, dissipate into the bulk sample due to heat conduction, and remain in the sample as residual thermal energy. The remaining energy fol-
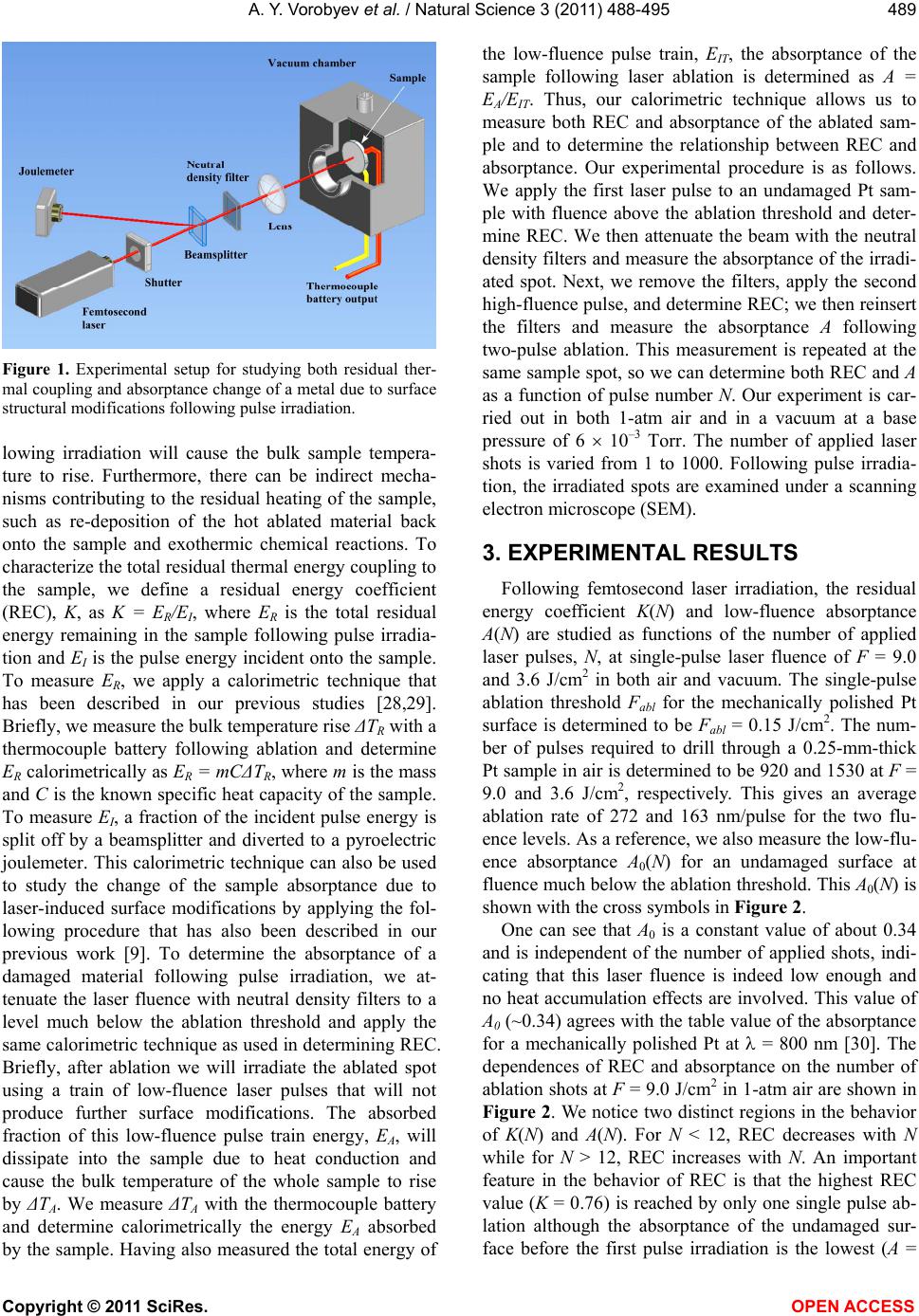 A. Y. Vorobyev et al. / Natural Science 3 (2011) 488-495 Copyright © 2011 SciRes. OPEN ACCESS 489 Figure 1. Experimental setup for studying both residual ther- mal coupling and absorptance change of a metal due to surface structural modifications following pulse irradiation. lowing irradiation will cause the bulk sample tempera- ture to rise. Furthermore, there can be indirect mecha- nisms contributing to the residual heating of the sample, such as re-deposition of the hot ablated material back onto the sample and exothermic chemical reactions. To characterize the total residual thermal energy coupling to the sample, we define a residual energy coefficient (REC), K, as K = ER/EI, where ER is the total residual energy remaining in the sample following pulse irradia- tion and EI is the pulse energy incident onto the sample. To measure ER, we apply a calorimetric technique that has been described in our previous studies [28,29]. Briefly, we measure the bulk temperature rise ΔTR with a thermocouple battery following ablation and determine ER calorimetrically as ER = mCΔTR, where m is the mass and C is the known specific heat capacity of the sample. To measure EI, a fraction of the incident pulse energy is split off by a beamsplitter and diverted to a pyroelectric joulemeter. This calorimetric technique can also be used to study the change of the sample absorptance due to laser-induced surface modifications by applying the fol- lowing procedure that has also been described in our previous work [9]. To determine the absorptance of a damaged material following pulse irradiation, we at- tenuate the laser fluence with neutral density filters to a level much below the ablation threshold and apply the same calorimetric technique as used in determining REC. Briefly, after ablation we will irradiate the ablated spot using a train of low-fluence laser pulses that will not produce further surface modifications. The absorbed fraction of this low-fluence pulse train energy, EA, will dissipate into the sample due to heat conduction and cause the bulk temperature of the whole sample to rise by ΔTA. We measure ΔTA with the thermocouple battery and determine calorimetrically the energy EA absorbed by the sample. Having also measured the total energy of the low-fluence pulse train, EIT, the absorptance of the sample following laser ablation is determined as A = EA/EIT. Thus, our calorimetric technique allows us to measure both REC and absorptance of the ablated sam- ple and to determine the relationship between REC and absorptance. Our experimental procedure is as follows. We apply the first laser pulse to an undamaged Pt sam- ple with fluence above the ablation threshold and deter- mine REC. We then attenuate the beam with the neutral density filters and measure the absorptance of the irradi- ated spot. Next, we remove the filters, apply the second high-fluence pulse, and determine REC; we then reinsert the filters and measure the absorptance A following two-pulse ablation. This measurement is repeated at the same sample spot, so we can determine both REC and A as a function of pulse number N. Our experiment is car- ried out in both 1-atm air and in a vacuum at a base pressure of 6 10–3 Torr. The number of applied laser shots is varied from 1 to 1000. Following pulse irradia- tion, the irradiated spots are examined under a scanning electron microscope (SEM). 3. EXPERIMENTAL RESULTS Following femtosecond laser irradiation, the residual energy coefficient K(N) and low-fluence absorptance A(N) are studied as functions of the number of applied laser pulses, N, at single-pulse laser fluence of F = 9.0 and 3.6 J/cm2 in both air and vacuum. The single-pulse ablation threshold Fabl for the mechanically polished Pt surface is determined to be Fabl = 0.15 J/cm2. The num- ber of pulses required to drill through a 0.25-mm-thick Pt sample in air is determined to be 920 and 1530 at F = 9.0 and 3.6 J/cm2, respectively. This gives an average ablation rate of 272 and 163 nm/pulse for the two flu- ence levels. As a reference, we also measure the low-flu- ence absorptance A0(N) for an undamaged surface at fluence much below the ablation threshold. This A0(N) is shown with the cross symbols in Figure 2. One can see that A0 is a constant value of about 0.34 and is independent of the number of applied shots, indi- cating that this laser fluence is indeed low enough and no heat accumulation effects are involved. This value of A0 (~0.34) agrees with the table value of the absorptance for a mechanically polished Pt at = 800 nm [30]. The dependences of REC and absorptance on the number of ablation shots at F = 9.0 J/cm2 in 1-atm air are shown in Figure 2. We notice two distinct regions in the behavior of K(N) and A(N). For N < 12, REC decreases with N while for N > 12, REC increases with N. An important feature in the behavior of REC is that the highest REC value (K = 0.76) is reached by only one single pulse ab- lation although the absorptance of the undamaged sur- face before the first pulse irradiation is the lowest (A =
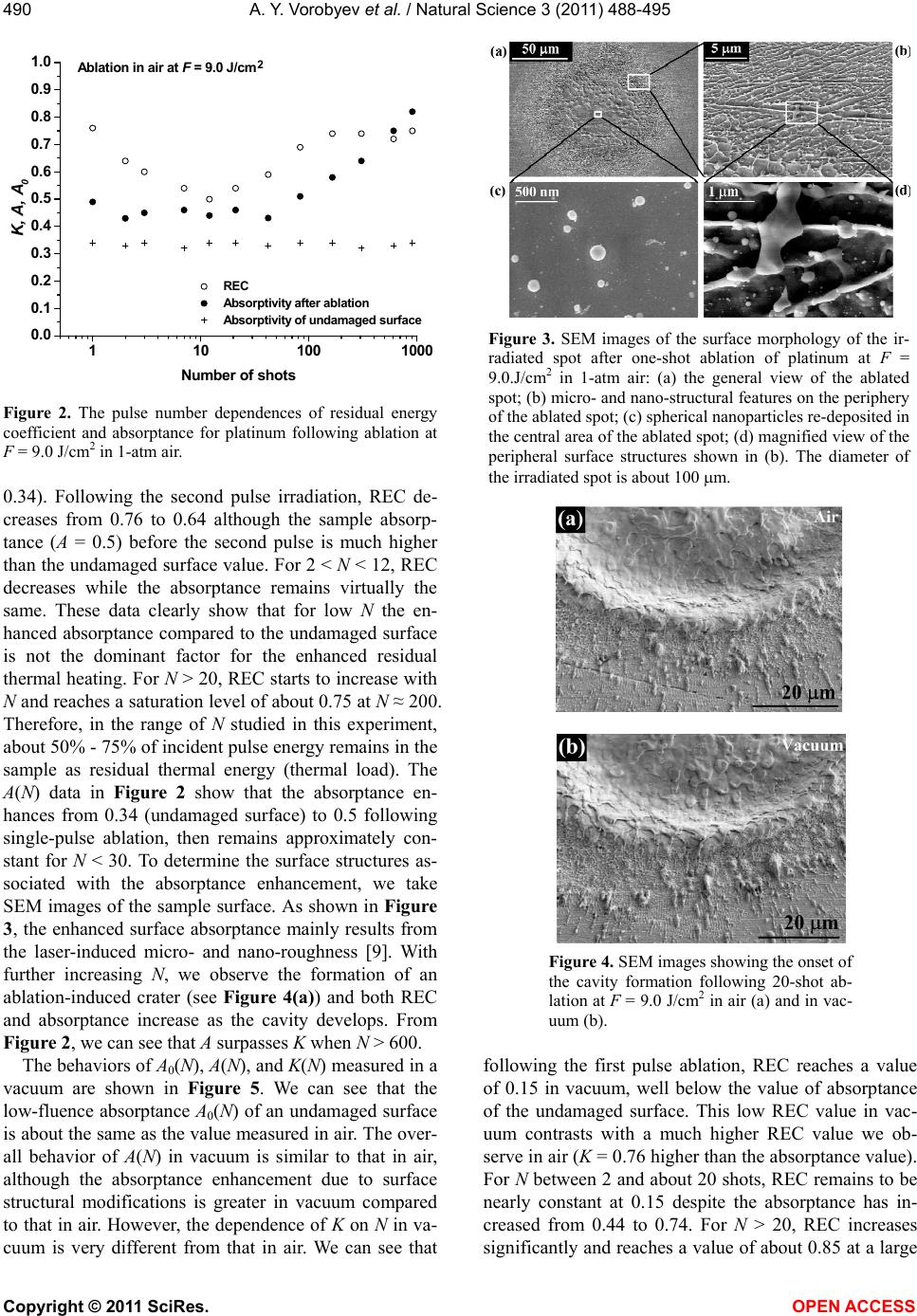 A. Y. Vorobyev et al. / Natural Science 3 (2011) 488-495 Copyright © 2011 SciRes. OPEN ACCESS 490 110100 1000 0.0 0.1 0.2 0.3 0.4 0.5 0.6 0.7 0.8 0.9 1.0 2 Ablation in air at F = 9.0 J/cm REC Absorptivity after ablation Absorptivity of undamaged surface K, A, A0 Number of shots Figure 2. The pulse number dependences of residual energy coefficient and absorptance for platinum following ablation at F = 9.0 J/cm2 in 1-atm air. 0.34). Following the second pulse irradiation, REC de- creases from 0.76 to 0.64 although the sample absorp- tance (A = 0.5) before the second pulse is much higher than the undamaged surface value. For 2 < N < 12, REC decreases while the absorptance remains virtually the same. These data clearly show that for low N the en- hanced absorptance compared to the undamaged surface is not the dominant factor for the enhanced residual thermal heating. For N > 20, REC starts to increase with N and reaches a saturation level of about 0.75 at N ≈ 200. Therefore, in the range of N studied in this experiment, about 50% - 75% of incident pulse energy remains in the sample as residual thermal energy (thermal load). The A(N) data in Figure 2 show that the absorptance en- hances from 0.34 (undamaged surface) to 0.5 following single-pulse ablation, then remains approximately con- stant for N < 30. To determine the surface structures as- sociated with the absorptance enhancement, we take SEM images of the sample surface. As shown in Figure 3, the enhanced surface absorptance mainly results from the laser-induced micro- and nano-roughness [9]. With further increasing N, we observe the formation of an ablation-induced crater (see Figure 4(a)) and both REC and absorptance increase as the cavity develops. From Figure 2, we can see that A surpasses K when N > 600. The behaviors of A0(N), A(N), and K(N) measured in a vacuum are shown in Figure 5. We can see that the low-fluence absorptance A0(N) of an undamaged surface is about the same as the value measured in air. The over- all behavior of A(N) in vacuum is similar to that in air, although the absorptance enhancement due to surface structural modifications is greater in vacuum compared to that in air. However, the dependence of K on N in va- cuum is very different from that in air. We can see that Figure 3. SEM images of the surface morphology of the ir- radiated spot after one-shot ablation of platinum at F = 9.0.J/cm2 in 1-atm air: (a) the general view of the ablated spot; (b) micro- and nano-structural features on the periphery of the ablated spot; (c) spherical nanoparticles re-deposited in the central area of the ablated spot; (d) magnified view of the peripheral surface structures shown in (b). The diameter of the irradiated spot is about 100 m. Figure 4. SEM images showing the onset of the cavity formation following 20-shot ab- lation at F = 9.0 J/cm2 in air (a) and in vac- uum (b). following the first pulse ablation, REC reaches a value of 0.15 in vacuum, well below the value of absorptance of the undamaged surface. This low REC value in vac- uum contrasts with a much higher REC value we ob- serve in air (K = 0.76 higher than the absorptance value). For N between 2 and about 20 shots, REC remains to be nearly constant at 0.15 despite the absorptance has in- creased from 0.44 to 0.74. For N > 20, REC increases significantly and reaches a value of about 0.85 at a large
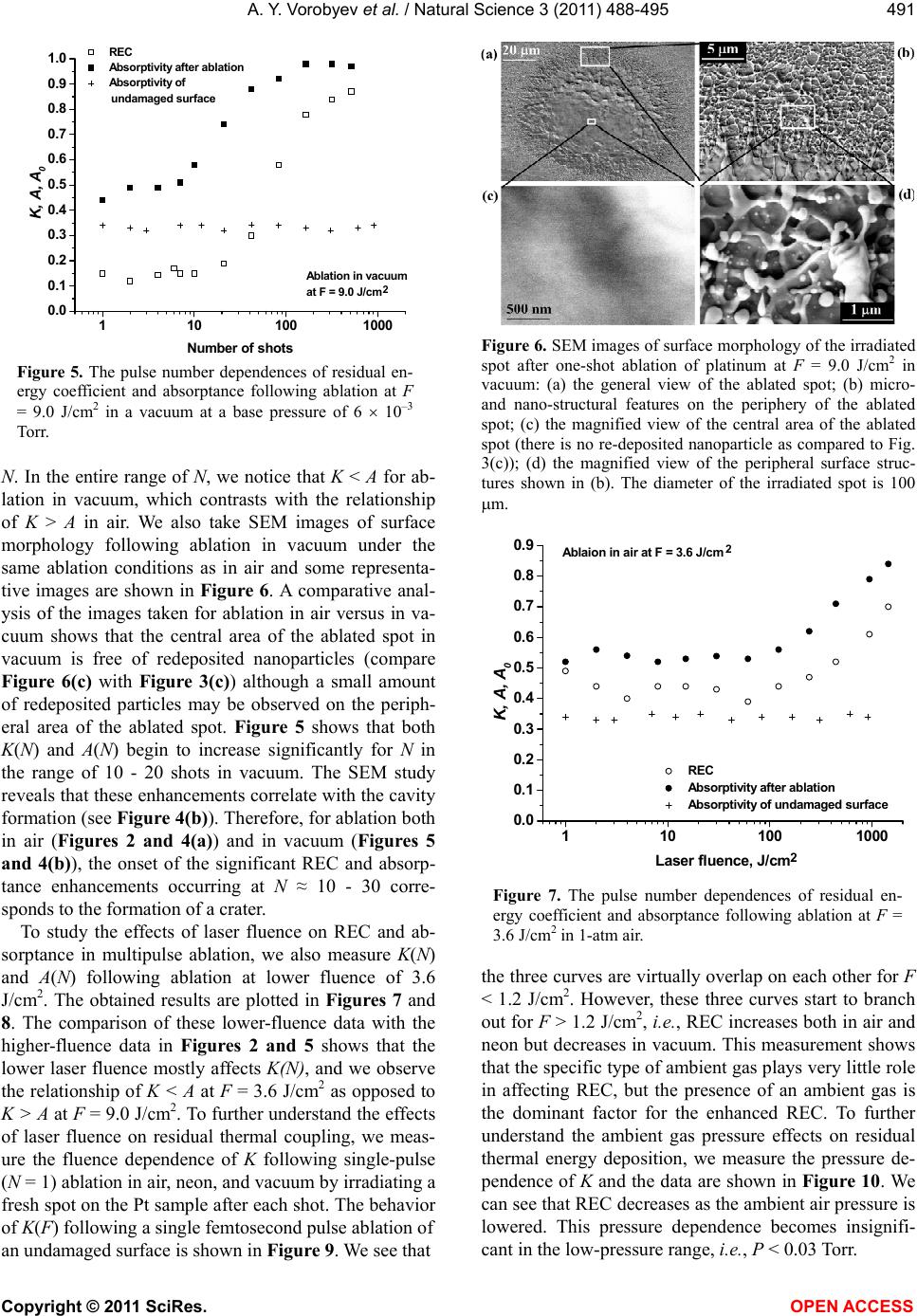 A. Y. Vorobyev et al. / Natural Science 3 (2011) 488-495 Copyright © 2011 SciRes. OPEN ACCESS 491 110100 1000 0.0 0.1 0.2 0.3 0.4 0.5 0.6 0.7 0.8 0.9 1.0 2 Ablation in vacuum at F = 9.0 J/cm REC Absorptivity after ablation Absorptivity of undamaged surface K, A, A0 Number of shots Figure 5. The pulse number dependences of residual en- ergy coefficient and absorptance following ablation at F = 9.0 J/cm2 in a vacuum at a base pressure of 6 10–3 Torr. N. In the entire range of N, we notice that K < A for ab- lation in vacuum, which contrasts with the relationship of K > A in air. We also take SEM images of surface morphology following ablation in vacuum under the same ablation conditions as in air and some representa- tive images are shown in Figure 6. A comparative anal- ysis of the images taken for ablation in air versus in va- cuum shows that the central area of the ablated spot in vacuum is free of redeposited nanoparticles (compare Figure 6(c) with Figure 3(c)) although a small amount of redeposited particles may be observed on the periph- eral area of the ablated spot. Figure 5 shows that both K(N) and A(N) begin to increase significantly for N in the range of 10 - 20 shots in vacuum. The SEM study reveals that these enhancements correlate with the cavity formation (see Figure 4(b)). Therefore, for ablation both in air (Figures 2 and 4(a)) and in vacuum (Figures 5 and 4(b)), the onset of the significant REC and absorp- tance enhancements occurring at N ≈ 10 - 30 corre- sponds to the formation of a crater. To study the effects of laser fluence on REC and ab- sorptance in multipulse ablation, we also measure K(N) and A(N) following ablation at lower fluence of 3.6 J/cm2. The obtained results are plotted in Figures 7 and 8. The comparison of these lower-fluence data with the higher-fluence data in Figures 2 and 5 shows that the lower laser fluence mostly affects K(N), and we observe the relationship of K < A at F = 3.6 J/cm2 as opposed to K > A at F = 9.0 J/cm2. To further understand the effects of laser fluence on residual thermal coupling, we meas- ure the fluence dependence of K following single-pulse (N = 1) ablation in air, neon, and vacuum by irradiating a fresh spot on the Pt sample after each shot. The behavior of K(F) following a single femtosecond pulse ablation of an undamaged surface is shown in Figure 9. We see that Figure 6. SEM images of surface morphology of the irradiated spot after one-shot ablation of platinum at F = 9.0 J/cm2 in vacuum: (a) the general view of the ablated spot; (b) micro- and nano-structural features on the periphery of the ablated spot; (c) the magnified view of the central area of the ablated spot (there is no re-deposited nanoparticle as compared to Fig. 3(c)); (d) the magnified view of the peripheral surface struc- tures shown in (b). The diameter of the irradiated spot is 100 m. 110100 1000 0.0 0.1 0.2 0.3 0.4 0.5 0.6 0.7 0.8 0.9 2 Ablaion in air at F = 3.6 J/cm 2 REC Absorptivity after ablation Absorptivity of undamaged surface K, A, A0 Laser fluence, J/cm Figure 7. The pulse number dependences of residual en- ergy coefficient and absorptance following ablation at F = 3.6 J/cm2 in 1-atm air. the three curves are virtually overlap on each other for F < 1.2 J/cm2. However, these three curves start to branch out for F > 1.2 J/cm2, i.e., REC increases both in air and neon but decreases in vacuum. This measurement shows that the specific type of ambient gas plays very little role in affecting REC, but the presence of an ambient gas is the dominant factor for the enhanced REC. To further understand the ambient gas pressure effects on residual thermal energy deposition, we measure the pressure de- pendence of K and the data are shown in Figure 10. We can see that REC decreases as the ambient air pressure is lowered. This pressure dependence becomes insignifi- cant in the low-pressure range, i.e., P < 0.03 Torr.
 A. Y. Vorobyev et al. / Natural Science 3 (2011) 488-495 Copyright © 2011 SciRes. OPEN ACCESS 492 110100 1000 0.0 0.1 0.2 0.3 0.4 0.5 0.6 0.7 0.8 0.9 1.0 2 Ablation in vacuum at F = 3.6 J/cm REC Absorptivity after ablation K, A Number of shots Figure 8. The pulse number dependences of residual energy coefficient and absorptance following ablation at F = 3.6 J/cm2 in a vacuum at a base pressure of 6 10–3 Torr. 0.01 0.1110 0.0 0.1 0.2 0.3 0.4 0.5 0.6 0.7 0.8 0.9 1.0 2 Ablation threshold 1-atm air Vacuum 1.08-atm Ne Residual energy coefficient Laser fluence, J/cm Figure 9. Residual energy coefficient for platinum as a func- tion of laser fluence in 1-atm air, 1.08-atm Ne, and a vacuum at a base pressure of 6 10–3 Torr. 0.010.1110100 1000 0.1 0.2 0.3 0.4 0.5 0.6 0.7 0.8 0.9 1.0 2 F = 10 J/cm Residual energyu coefficient Air pressure, Torr Figure 10. Residual energy coefficient for platinum as a function of air pressure at F = 10 J/cm2. 4. DISCUSSIONS Thermal response of metals following high-fluence laser irradiation has been studied in the past using mi- crosecond [31] and nanosecond [32] laser pulses, and enhanced heat deposition in metals has been found in those experiments when plasmas are generated. To ex- plain those enhanced thermal energy coupling, it has been suggested that energy transferring to a metal sam- ple from laser-induced plasmas plays a key role [31]. Previously, we have found that the behavior of residual thermal energy coupling shows a similar general trend between nanosecond- and femtosecond-laser pulse abla- tion [28], and this suggests that the physical mechanism used to explain the long-pulse REC enhancement may also account for the behaviors of residual heating in femtosecond laser ablation. In general, two types of plas- mas can be produced during a femtosecond laser pulse: 1) solid-density plasma in the skin layer of the sample and 2) ambient gas plasma. Solid-density plasma can be produced in both air and vacuum; in our experiment, however, the enhanced thermal coupling occurs only in air and therefore, we believe the solid-density plasma does not play a key role in the enhanced energy coupling. Therefore, we will subsequently focus our discussion on the effects of ambient air plasma. The experimental data shown in Figure 2 are obtained with an intensity of about 1014 W/cm2. At this high in- tensity, direct ionization of the ambient air during the femtosecond laser pulse easily occurs by means of mul- tiphoton and tunneling ionization even without the pres- ence of a metal sample [33]. In the presence of a metal surface, the threshold of air ionization in front of the metal surface can be significantly reduced due to: 1) air exposing to the additional laser light reflected from the metal surface besides the incident light [34], 2) energetic electrons escaping from the metal surface due to mul- tiphoton photoelectron and thermoionic emission [35], and 3) UV radiation from laser-induced solid-density plasma, if any. The above three processes may addition- ally contribute to air ionization besides the dominant multiphoton ionization [34] from the incident laser light. It should be emphasized that this air plasma is produced prior to the hydrodynamic motion of the ablated material from the sample that occurs on the nanosecond time scale [36,37]. We note that the generation of air plasma prior to the hydrodynamic motion of the ablated material has been observed in the past in picosecond laser abla- tion [38]. The difference between plasmas produced in air and in vacuum can clearly be seen from the plasma plume images in Figure 11, which are taken using an open-shutter camera [28]. We can see that the size of hot plasma generated following ablation in air is larger than that in vacuum. Since material ejection in vacuum is not
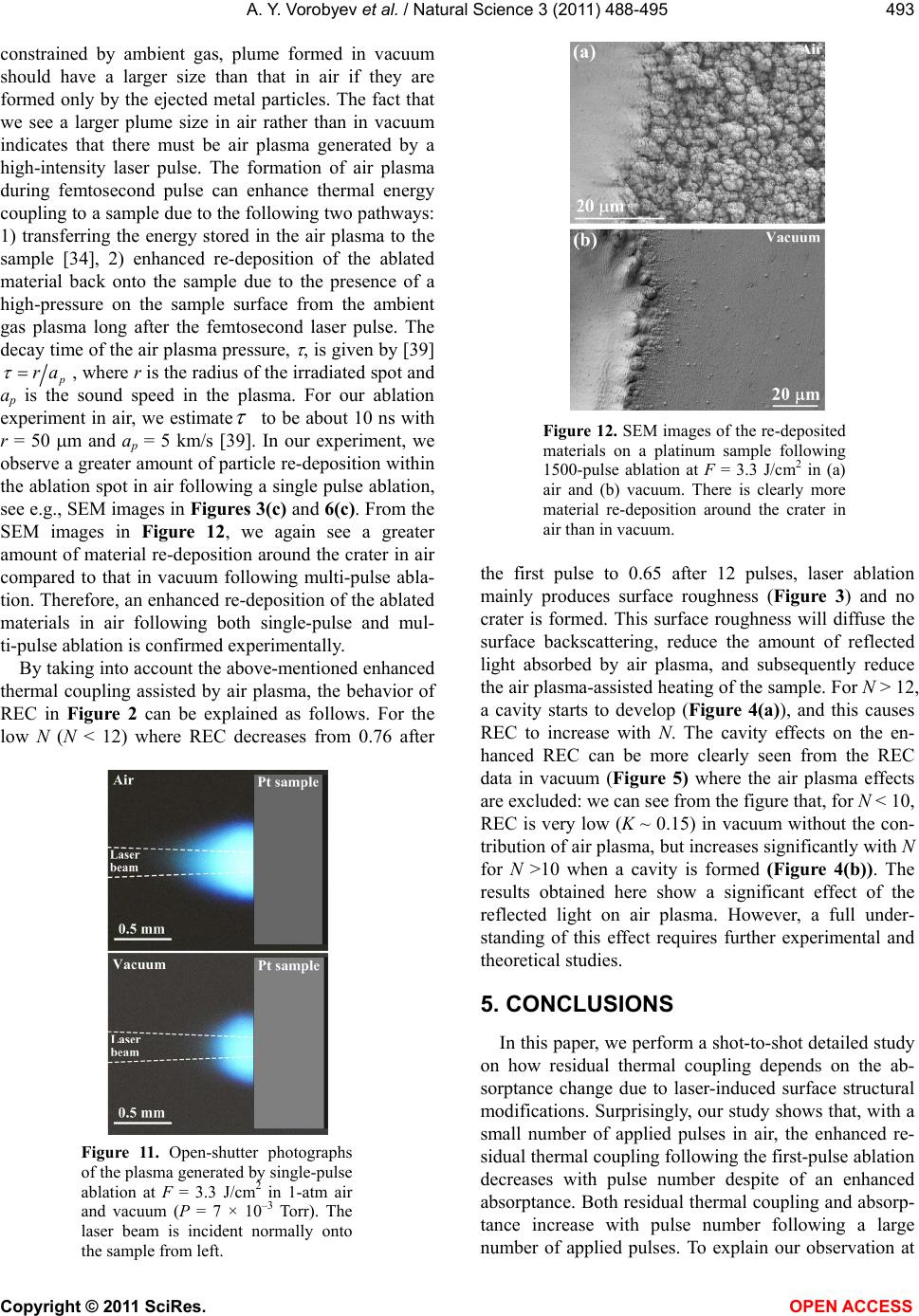 A. Y. Vorobyev et al. / Natural Science 3 (2011) 488-495 Copyright © 2011 SciRes. OPEN ACCESS 493 constrained by ambient gas, plume formed in vacuum should have a larger size than that in air if they are formed only by the ejected metal particles. The fact that we see a larger plume size in air rather than in vacuum indicates that there must be air plasma generated by a high-intensity laser pulse. The formation of air plasma during femtosecond pulse can enhance thermal energy coupling to a sample due to the following two pathways: 1) transferring the energy stored in the air plasma to the sample [34], 2) enhanced re-deposition of the ablated material back onto the sample due to the presence of a high-pressure on the sample surface from the ambient gas plasma long after the femtosecond laser pulse. The decay time of the air plasma pressure, , is given by [39] ra , where r is the radius of the irradiated spot and ap is the sound speed in the plasma. For our ablation experiment in air, we estimate to be about 10 ns with r = 50 m and ap = 5 km/s [39]. In our experiment, we observe a greater amount of particle re-deposition within the ablation spot in air following a single pulse ablation, see e.g., SEM images in Figures 3(c) and 6(c). From the SEM images in Figure 12, we again see a greater amount of material re-deposition around the crater in air compared to that in vacuum following multi-pulse abla- tion. Therefore, an enhanced re-deposition of the ablated materials in air following both single-pulse and mul- ti-pulse ablation is confirmed experimentally. By taking into account the above-mentioned enhanced thermal coupling assisted by air plasma, the behavior of REC in Figure 2 can be explained as follows. For the low N (N < 12) where REC decreases from 0.76 after Figure 11. Open-shutter photographs of the plasma generated by single-pulse ablation at F = 3.3 J/cm2 in 1-atm air and vacuum (P = 7 × 10–3 Torr). The laser beam is incident normally onto the sample from left. Figure 12. SEM images of the re-deposited materials on a platinum sample following 1500-pulse ablation at F = 3.3 J/cm2 in (a) air and (b) vacuum. There is clearly more material re-deposition around the crater in air than in vacuum. the first pulse to 0.65 after 12 pulses, laser ablation mainly produces surface roughness (Figure 3) and no crater is formed. This surface roughness will diffuse the surface backscattering, reduce the amount of reflected light absorbed by air plasma, and subsequently reduce the air plasma-assisted heating of the sample. For N > 12, a cavity starts to develop (Figure 4(a)), and this causes REC to increase with N. The cavity effects on the en- hanced REC can be more clearly seen from the REC data in vacuum (Figure 5) where the air plasma effects are excluded: we can see from the figure that, for N < 10, REC is very low (K ~ 0.15) in vacuum without the con- tribution of air plasma, but increases significantly with N for N >10 when a cavity is formed (Figure 4(b)). The results obtained here show a significant effect of the reflected light on air plasma. However, a full under- standing of this effect requires further experimental and theoretical studies. 5. CONCLUSIONS In this paper, we perform a shot-to-shot detailed study on how residual thermal coupling depends on the ab- sorptance change due to laser-induced surface structural modifications. Surprisingly, our study shows that, with a small number of applied pulses in air, the enhanced re- sidual thermal coupling following the first-pulse ablation decreases with pulse number despite of an enhanced absorptance. Both residual thermal coupling and absorp- tance increase with pulse number following a large number of applied pulses. To explain our observation at
 A. Y. Vorobyev et al. / Natural Science 3 (2011) 488-495 Copyright © 2011 SciRes. OPEN ACCESS 494 a low pulse number, we propose a plasma-assisted me- chanism for femtosecond laser ablation in a gas medium. Supported by our experimental results, we believe that the formation of ambient gas plasma enhances thermal energy coupling to a sample due to the following two pathways: energy transfer from the air plasma to the sample and enhanced re-deposition of hot ablated parti- cles back onto the sample due to the presence of high pressure from the ambient gas plasma. These plas- ma-assisted effects can only play a role in a gas medium and are most important following a relatively low num- ber of applied shots when only surface roughness but not a crater develops. At a large number of applied pulses, the residual heating of the sample is mainly governed by the formation of craters that enhance thermal energy coupling to the sample both in air and in vacuum. Al- though further studies are needed to better understand the physical mechanisms of thermal energy coupling to solids, our study provides new guidelines to determine the optimal thermal-loading conditions for achieving high-quality material processing using femtosecond laser pulses. 6. ACKNOWLEDGMENTS The authors acknowledge R. Grzegorzak for assistance with vacuum equipment. The research was supported by the National Science Foun- dation and US Air Force Office of Scientific Research. REFERENCES [1] Pronko, P.P., Dutta, S.K., Squier, J., Rudd, J.V., Du, D. and Mourou G. (1995) Machining of sub-micron holes using a femtosecond laser at 800 nm. Optics Communi- cations, 114 , 106-110. doi:10.1016/0030-4018(94)00585-I [2] Chichkov, B. N., Momma, C., Nolte, S., von Alvensleben, F. and Tunnermann, A. (1996) Femtosecond, picosecond and nanosecond laser ablation of solids. Applied Physics A, 63, 109-115. doi:10.1007/BF01567637 [3] Pereira, A., Cros, A., Delaporte, P., Georgiou, S., Ma- nousaki, A., Marine, W. and Sentis, M. (2004) Surface nanostructuring of metals by laser irradiation: Effects of pulse duration, wavelength and gas atmosphere. Applied Physics A, 79, 1433-1437. doi:10.1007/s00339-004-2804-x [4] Nolte, S., Chichkov, B.N., Welling, H., Shani, Y., Lie- bermann, K. and Terkel, H. (1999) Nanostructuring with spatially localized femtosecond laser pulses. Optics Let- ters, 24, 914-916. doi:10.1364/OL.24.000914 [5] Amoruso, S., Ausanio, G., Bruzzese, R., Vitello, M. and Wang, X. (2005) Femtosecond laser pulse irradiation of solid targets as a general route to nanoparticle formation in a vacuum. Physical Review B, 71, 033406. doi:10.1103/PhysRevB.71.033406 [6] Koch, J., Korte, F., Bauer, T., Fallnich, C., Ostendorf, A. and Chichkov, B.N. (2005) Nanotexturing of gold films by femtosecond laser-induced melt dynamics. Applied Physics A, 81, 325-328. doi:10.1007/s00339-005-3212-6 [7] Vorobyev, A.Y. and Guo, C. (2006) Femtosecond laser nanostructuring of metals. Optics Express, 14, 2164-2169. doi:10.1364/OE.14.002164 [8] Wu, C., Crouch, C.H., Zhao, L., Carey, J.E., Younkin, R., Levinson, J.A., Mazur, E., Farrell, R.M., Gothoskar, P. and Karger, A. (2001) Near-unity below-band-gap ab- sorption by microstructured silicon. Applied Physics Let- ters, 78, 1850-1852. doi:10.1063/1.1358846 [9] Vorobyev, A.Y. and Guo, C. (2005) Enhanced absorp- tance of gold following multi-pulse femtosecond laser ablation. Physical Review B, 72, 195422. doi:10.1103/PhysRevB.72.195422 [10] Vorobyev, A.Y. and Guo, C. (2008) Colorizing metals with femtosecond laser pulses. Applied Physics Letters, 92, 041914. doi:10.1063/1.2834902 [11] Kaakkunen, J.J.J., Paivasaari, K., Kuittinen, M. and Jaaskelainen, T. (2009) Morphology studies of the metal surfaces with enhanced absorption fabricated using in- terferometric femtosecond ablation. Applied Physics A, 94, 215-220. doi:10.1007/s00339-008-4895-2 [12] Paivasaari, K., Kaakkunen, J.J.J., Kuittinen, M. and Jaaskelainen, T. (2007) Enhanced optical absorptance of metals using interferometric femtosecond ablation. Op- tics Express, 15, 13838-13843. doi:10.1364/OE.15.013838 [13] Ausano, G., Barone, A.C., Iannotti, V., Lanotte, L., Amo- ruso, S., Bruzzese, R. and Vitiello M. (2004) Magnetic and morphological characteristics of nickel nanoparticles films produced by femtosecond laser ablation. Applied Physics Letters, 85, 4103-4105. doi:10.1063/1.1815065 [14] Baldacchini, T., Carey, J.E., Zhou, M. and Mazur, E. (2006) Superhydrophobic Surfaces Prepared by Micro- structuring of Silicon Using a Femtosecond Laser. Langmuir, 22, 4917-4919. doi:10.1021/la053374k [15] Zorba, V., Persano, L., Pisignano, D., Athanassiou, A., Stratakis, E., Cingolani, R., Tzanetakis, P. and Fotakis, C. (2006) Making silicon hydrophobic: Wettability control by two-lengthscale simultaneous patterning with femto- second laser irradiation. Nanotechnology, 17, 3234-3238. doi:10.1088/0957-4484/17/13/026 [16] Vorobyev, A.Y. and Guo, C. (2009) Metal pumps liquid uphill. Applied Physics Letters, 94, 224102. doi:10.1063/1.3117237 [17] Fadeeva, E., Schlie, S., Koch, J., Chichkov, B.N., Vo- robyev, A.Y. and Guo, C. (2009) Femtosecond laser-in- duced surface structures on platinum and their effects on hydrophobicity and fibroblast cell proliferation. Contact Angle, Wettability and Adhesion, 6, 163-171. [18] Qian, F., Cracuin, V., Singh, R.K., Dutta, S.D. and Pron- ko, P.P. (1999) High intensity femtosecond laser deposi- tion of diamond-like carbon films. Journal of Applied Physics, 86, 2281-2290. doi:10.1063/1.371043 [19] Luke, J.R., Phipps, C.R. and McDuff, G.G. (2003) Laser plasma thruster. Applied Physics A, 77, 343-348. [20] Polte, T.R., Shen, M., Karavitis, J., Montoya, M., Pendse, J., Xia, S., Mazur, E. and Ingber, D.E. (2007) Nanostruc- tured magnetizeable materials that switch cells between life and death. Biomaterials, 28, 2783-2790. doi:10.1016/j.biomaterials.2007.01.045 [21] Anisimov, S.I. and Luk'yanchuk, B.S. (2002) Selected
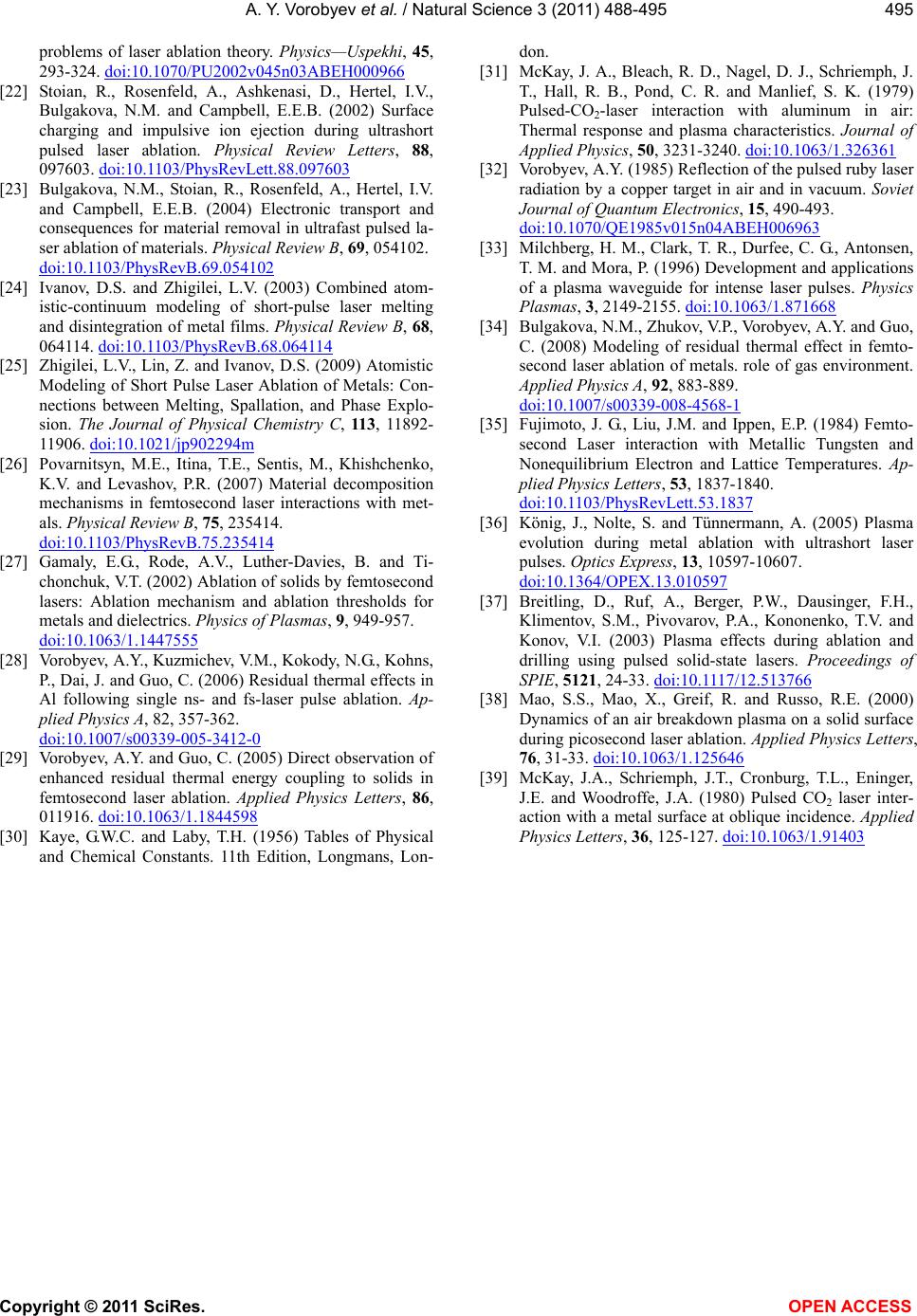 A. Y. Vorobyev et al. / Natural Science 3 (2011) 488-495 Copyright © 2011 SciRes. OPEN ACCESS 495 problems of laser ablation theory. Physics—Uspekhi, 45, 293-324. doi:10.1070/PU2002v045n03ABEH000966 [22] Stoian, R., Rosenfeld, A., Ashkenasi, D., Hertel, I.V., Bulgakova, N.M. and Campbell, E.E.B. (2002) Surface charging and impulsive ion ejection during ultrashort pulsed laser ablation. Physical Review Letters, 88, 097603. doi:10.1103/Phy sRevLett.88.097603 [23] Bulgakova, N.M., Stoian, R., Rosenfeld, A., Hertel, I.V. and Campbell, E.E.B. (2004) Electronic transport and consequences for material removal in ultrafast pulsed la- ser ablation of materials. Physical Review B, 69, 054102. doi:10.1103/PhysRevB.69.054102 [24] Ivanov, D.S. and Zhigilei, L.V. (2003) Combined atom- istic-continuum modeling of short-pulse laser melting and disintegration of metal films. Physical Review B, 68, 064114. doi:10.1103/PhysRevB.68.064114 [25] Zhigilei, L.V., Lin, Z. and Ivanov, D.S. (2009) Atomistic Modeling of Short Pulse Laser Ablation of Metals: Con- nections between Melting, Spallation, and Phase Explo- sion. The Journal of Physical Chemistry C, 113, 11892- 11906. doi:10.1021/jp902294m [26] Povarnitsyn, M.E., Itina, T.E., Sentis, M., Khishchenko, K.V. and Levashov, P.R. (2007) Material decomposition mechanisms in femtosecond laser interactions with met- als. Physical Review B, 75, 235414. doi:10.1103/PhysRevB.75.235414 [27] Gamaly, E.G., Rode, A.V., Luther-Davies, B. and Ti- chonchuk, V.T. (2002) Ablation of solids by femtosecond lasers: Ablation mechanism and ablation thresholds for metals and dielectrics. Physics of Plasmas, 9, 949-957. doi:10.1063/1.1447555 [28] Vorobyev, A.Y., Kuzmichev, V.M., Kokody, N.G., Kohns, P., Dai, J. and Guo, C. (2006) Residual thermal effects in Al following single ns- and fs-laser pulse ablation. Ap- plied Physics A, 82, 357-362. doi:10.1007/s00339-005-3412-0 [29] Vorobyev, A.Y. and Guo, C. (2005) Direct observation of enhanced residual thermal energy coupling to solids in femtosecond laser ablation. Applied Physics Letters, 86, 011916. doi:10.1063/1.1844598 [30] Kaye, G.W.C. and Laby, T.H. (1956) Tables of Physical and Chemical Constants. 11th Edition, Longmans, Lon- don. [31] McKay, J. A., Bleach, R. D., Nagel, D. J., Schriemph, J. T., Hall, R. B., Pond, C. R. and Manlief, S. K. (1979) Pulsed-CO2-laser interaction with aluminum in air: Thermal response and plasma characteristics. Journal of Applied Physics, 50, 3231-3240. doi:10.1063/1.326361 [32] Vorobyev, A.Y. (1985) Reflection of the pulsed ruby laser radiation by a copper target in air and in vacuum. Soviet Journal of Quantum Electronics, 15, 490-493. doi:10.1070/QE1985v015n04ABEH006963 [33] Milchberg, H. M., Clark, T. R., Durfee, C. G., Antonsen, T. M. and Mora, P. (1996) Development and applications of a plasma waveguide for intense laser pulses. Physics Plasmas, 3, 2149-2155. doi:10.1063/1.871668 [34] Bulgakova, N.M., Zhukov, V.P., Vorobyev, A.Y. and Guo, C. (2008) Modeling of residual thermal effect in femto- second laser ablation of metals. role of gas environment. Applied Physics A, 92, 883-889. doi:10.1007/s00339-008-4568-1 [35] Fujimoto, J. G., Liu, J.M. and Ippen, E.P. (1984) Femto- second Laser interaction with Metallic Tungsten and Nonequilibrium Electron and Lattice Temperatures. Ap- plied Physics Letters, 53, 1837-1840. doi:10.1103/PhysRevLett.53.1837 [36] König, J., Nolte, S. and Tünnermann, A. (2005) Plasma evolution during metal ablation with ultrashort laser pulses. Optics Express, 13, 10597-10607. doi:10.1364/OPEX.13.010597 [37] Breitling, D., Ruf, A., Berger, P.W., Dausinger, F.H., Klimentov, S.M., Pivovarov, P.A., Kononenko, T.V. and Konov, V.I. (2003) Plasma effects during ablation and drilling using pulsed solid-state lasers. Proceedings of SPIE, 5121, 24-33. doi:10.1117/12.513766 [38] Mao, S.S., Mao, X., Greif, R. and Russo, R.E. (2000) Dynamics of an air breakdown plasma on a solid surface during picosecond laser ablation. Applied Physics Letters, 76, 31-33. doi:10.1063/1.125646 [39] McKay, J.A., Schriemph, J.T., Cronburg, T.L., Eninger, J.E. and Woodroffe, J.A. (1980) Pulsed CO2 laser inter- action with a metal surface at oblique incidence. Applied Physics Letters, 36, 125-127. doi:10.1063/1.91403
|