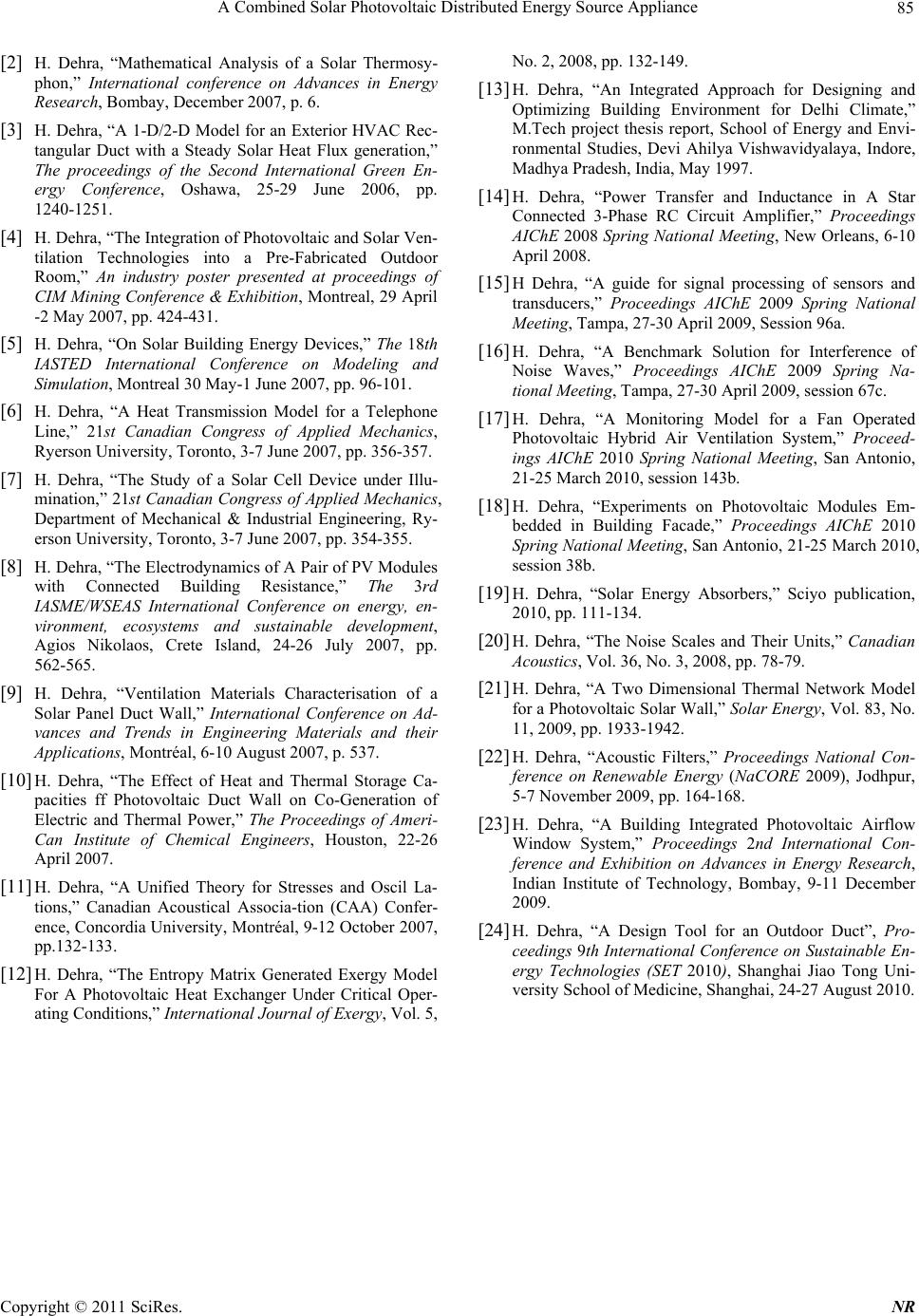
A Combined Solar Photovoltaic Distributed Energy Source Appliance 85
[2] H. Dehra, “Mathematical Analysis of a Solar Thermosy-
phon,” International conference on Advances in Energy
Research, Bombay, December 2007, p. 6.
[3] H. Dehra, “A 1-D/2-D Model for an Exterior HVAC Rec-
tangular Duct with a Steady Solar Heat Flux generation,”
The proceedings of the Second International Green En-
ergy Conference, Oshawa, 25-29 June 2006, pp.
1240-1251.
[4] H. Dehra, “The Integration of Photovoltaic and Solar Ven-
tilation Technologies into a Pre-Fabricated Outdoor
Room,” An industry poster presented at proceedings of
CIM Mining Conference & Exhibition, Montreal, 29 April
-2 May 2007, pp. 424-431.
[5] H. Dehra, “On Solar Building Energy Devices,” The 18th
IASTED International Conference on Modeling and
Simulation, Montreal 30 May-1 June 2007, pp. 96-101.
[6] H. Dehra, “A Heat Transmission Model for a Telephone
Line,” 21st Canadian Congress of Applied Mechanics,
Ryerson University, Toronto, 3-7 June 2007, pp. 356-357.
[7] H. Dehra, “The Study of a Solar Cell Device under Illu-
mination,” 21st Canadian Congress of Applied Mechanics,
Department of Mechanical & Industrial Engineering, Ry-
erson University, Toronto, 3-7 June 2007, pp. 354-355.
[8] H. Dehra, “The Electrodynamics of A Pair of PV Modules
with Connected Building Resistance,” The 3rd
IASME/WSEAS International Conference on energy, en-
vironment, ecosystems and sustainable development,
Agios Nikolaos, Crete Island, 24-26 July 2007, pp.
562-565.
[9] H. Dehra, “Ventilation Materials Characterisation of a
Solar Panel Duct Wall,” International Conference on Ad-
vances and Trends in Engineering Materials and their
Applications, Montréal, 6-10 August 2007, p. 537.
[10] H. Dehra, “The Effect of Heat and Thermal Storage Ca-
pacities ff Photovoltaic Duct Wall on Co-Generation of
Electric and Thermal Power,” The Proceedings of Ameri-
Can Institute of Chemical Engineers, Houston, 22-26
April 2007.
[11] H. Dehra, “A Unified Theory for Stresses and Oscil La-
tions,” Canadian Acoustical Associa-tion (CAA) Confer-
ence, Concordia University, Montréal, 9-12 October 2007,
pp.132-133.
[12] H. Dehra, “The Entropy Matrix Generated Exergy Model
For A Photovoltaic Heat Exchanger Under Critical Oper-
ating Conditions,” International Journal of Exergy, Vol. 5,
No. 2, 2008, pp. 132-149.
[13] H. Dehra, “An Integrated Approach for Designing and
Optimizing Building Environment for Delhi Climate,”
M.Tech project thesis report, School of Energy and Envi-
ronmental Studies, Devi Ahilya Vishwavidyalaya, Indore,
Madhya Pradesh, India, May 1997.
[14] H. Dehra, “Power Transfer and Inductance in A Star
Connected 3-Phase RC Circuit Amplifier,” Proceedings
AIChE 2008 Spring National Meeting, New Orleans, 6-10
April 2008.
[15] H Dehra, “A guide for signal processing of sensors and
transducers,” Proceedings AIChE 2009 Spring National
Meeting, Tampa, 27-30 April 2009, Session 96a.
[16] H. Dehra, “A Benchmark Solution for Interference of
Noise Waves,” Proceedings AIChE 2009 Spring Na-
tional Meeting, Tampa, 27-30 April 2009, session 67c.
[17] H. Dehra, “A Monitoring Model for a Fan Operated
Photovoltaic Hybrid Air Ventilation System,” Proceed-
ings AIChE 2010 Spring National Meeting, San Antonio,
21-25 March 2010, session 143b.
[18] H. Dehra, “Experiments on Photovoltaic Modules Em-
bedded in Building Facade,” Proceedings AIChE 2010
Spring National Meeting, San Antonio, 21-25 March 2010,
session 38b.
[19] H. Dehra, “Solar Energy Absorbers,” Sciyo publication,
2010, pp. 111-134.
[20] H. Dehra, “The Noise Scales and Their Units,” Canadian
Acoustics, Vol. 36, No. 3, 2008, pp. 78-79.
[21] H. Dehra, “A Two Dimensional Thermal Network Model
for a Photovoltaic Solar Wall,” Solar Energy, Vol. 83, No.
11, 2009, pp. 1933-1942.
[22] H. Dehra, “Acoustic Filters,” Proceedings National Con-
ference on Renewable Energy (NaCORE 2009), Jodhpur,
5-7 November 2009, pp. 164-168.
[23] H. Dehra, “A Building Integrated Photovoltaic Airflow
Window System,” Proceedings 2nd International Con-
ference and Exhibition on Advances in Energy Research,
Indian Institute of Technology, Bombay, 9-11 December
2009.
[24] H. Dehra, “A Design Tool for an Outdoor Duct”, Pro-
ceedings 9th International Conference on Sustainable En-
ergy Technologies (SET 2010), Shanghai Jiao Tong Uni-
versity School of Medicine, Shanghai, 24-27 August 2010.
Copyright © 2011 SciRes. NR