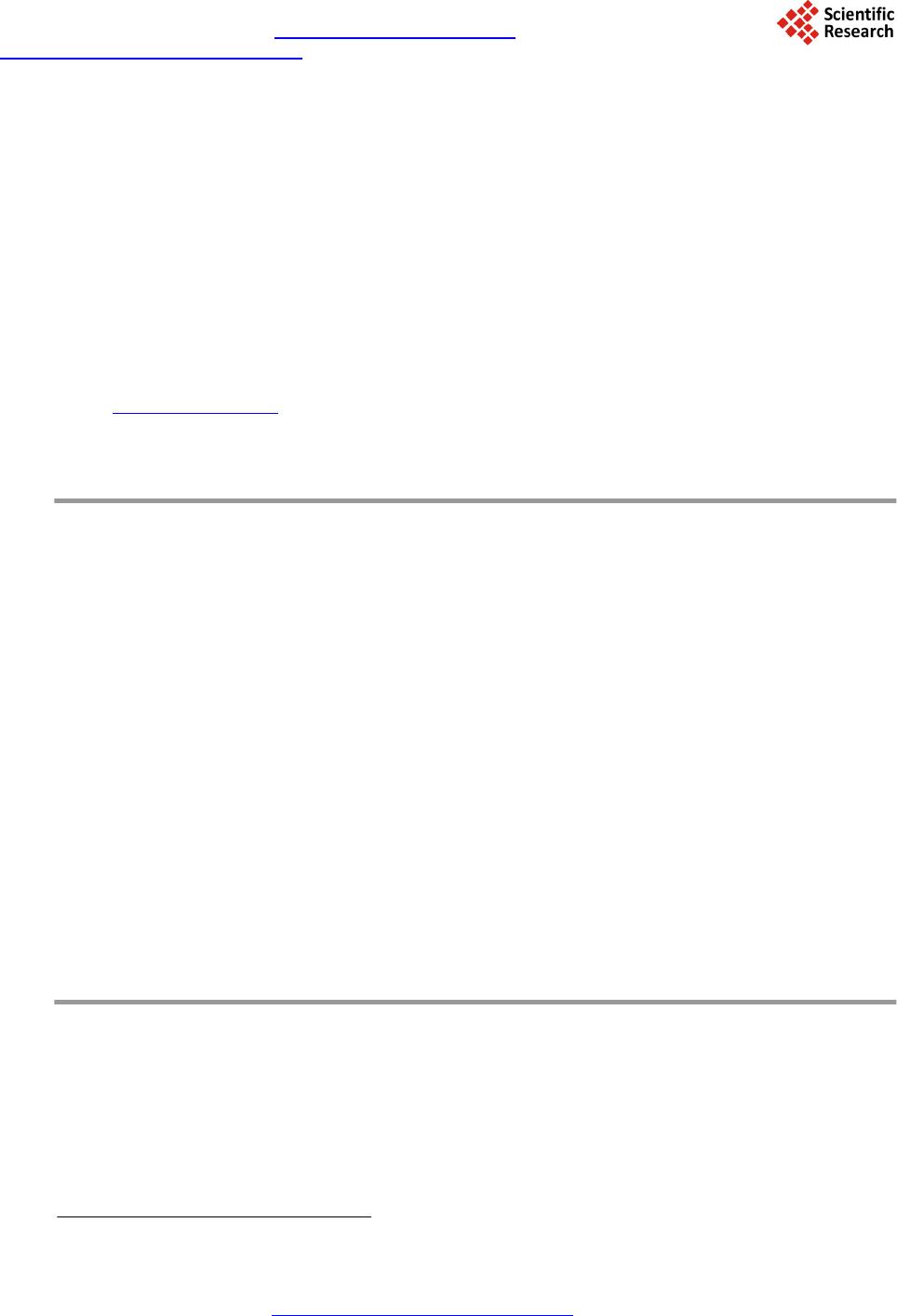
Journal of Applied Mathematics and Physics, 2014, 2, 910-917
Published Online August 2014 in SciRes. http://www.scirp.org/journal/jamp
http://dx.doi.org/10.4236/jamp.2014.29103
How to cite this paper: Hajdas, W., Desorgher, L., Deiters, K., Reggiani, D., Rauber, Th., Tulej, M., Wurz, P., Luethi, M.,
Wojczuk, K. and Kalaczynski, P. (2014) High Energy Electron Radiation Exposure Facility at PSI. Journal of Applied Mathe-
matics and Physics, 2, 910-917. http://dx.doi.org/10.4236/jamp.2014.29103
High Energy Electron Radiation Exposure
Facility at PSI
Wojtek Hajdas1*, L. Desorgher1, K. Deiters1, D. Reggiani1, Th. Rauber1, M. Tulej2, P. Wurz2,
M. Luethi2, K. Wojczuk1, P. Kalaczynski1
1Paul Scherrer Institut (PSI), Villigen, Switzerland
2University of Bern, Bern, Switzerland
Email: *wojtek.hajdas@psi.ch
Received 3 June 2014
Abstract
Paul Scherrer Institut hosts the Proton Irradiation Facility used for radiation effects studies and
exposure tests in preparation of satellite missions for the European Space Agency. The facility al-
lows for realistic simulation of the space proton spectra in the energy range from 6 MeV up to 230
MeV with exposure fluxes ranging from very low up to as high as 109 p/cm2/sec. Recently, ap-
proved ESA mission to Jupiter—JUICE—also brought a need for tests with high energy electron
beams. For this purpose, another facility was established in the PSI secondary beam area piM1.
Secondary particles are produced on the thick carbon target hit by energetic proton beam. Dedi-
cated beam optics enables selection of the particle charge and momentum and guides them to the
tests area. Characterization of electron beams at various momenta was performed with respect to
their intensity, profiles and contamination by pions and muons. Electron fluxes ranging from 1.5 ×
103/cm2/s at 20 MeV/c to 2.3 × 10 6/cm2/s at 345 MeV/c with gaussian beam profiles with FWHM
of about 4 cm were measured. Beam contamination with heavier particles becomes negligible for
all momenta lower than 115 MeV/c. This allows for using them for components and shielding cha-
racterization and detector calibration experiments. Several such experiments have been already
performed utilizing available beam time of few weeks per year.
Keywords
Irradiations, Electron Beams, Components Tests, Exposure Facilities
1. Introduction
Secondary beam experimental areas of PSI are mainly used for basic research tests with pions and muons. They
utilize 590 MeV, high intensity proton beam from the synchro-cyclotron and two carbon targets used to produce
secondary particles. Several beam lines were constructed and specialized in delivering of various secondary par-
ticles with respect to the type, charge and momentum. Design and complexity of each beam line influence such
beam parameters as particle intensity, range of its momenta and momentum resolution as well as contamination
*