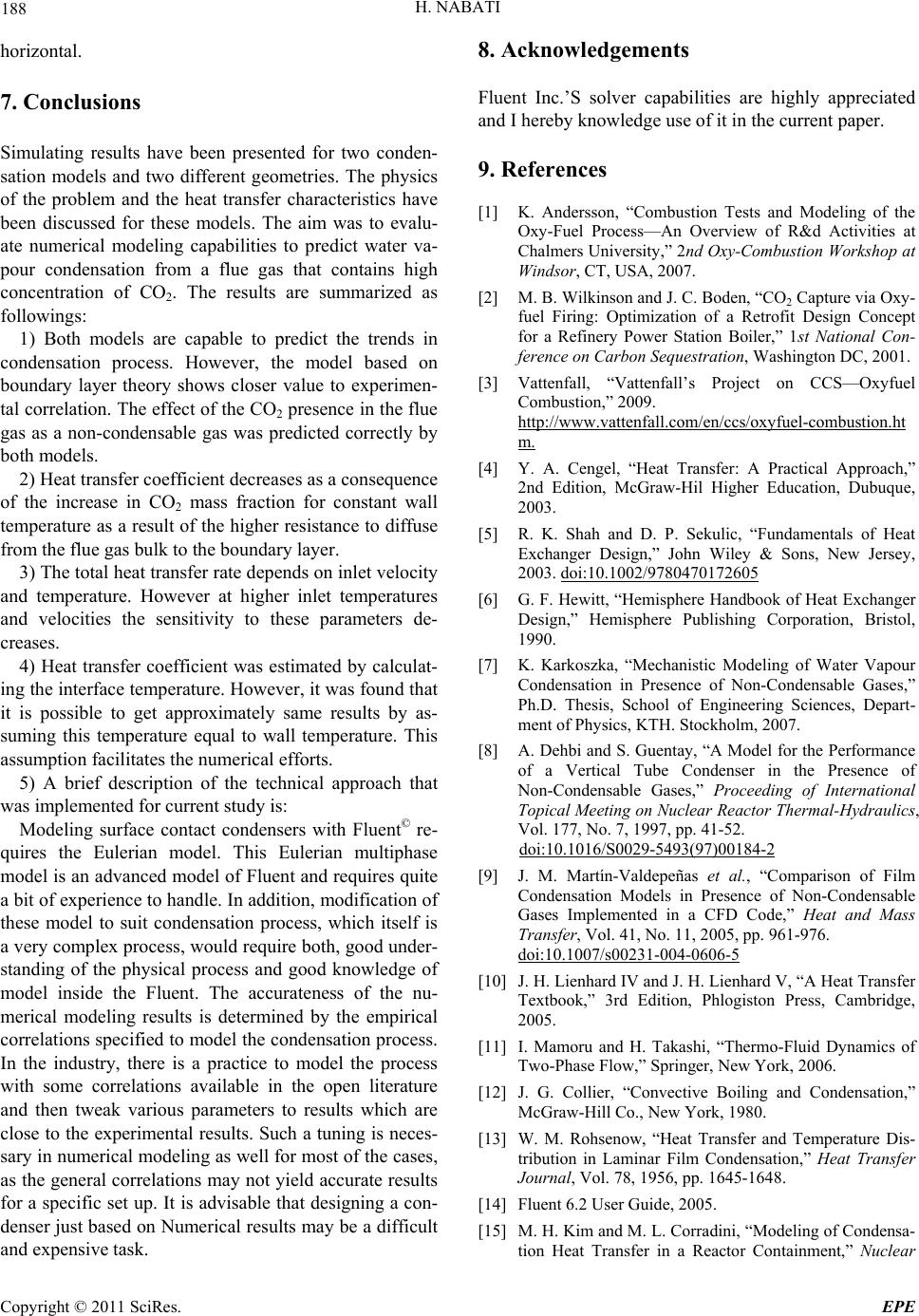
H. NABATI
188
horizontal.
7. Conclusions
Simulating results have been presented for two conden-
sation models and two different geometries. The physics
of the problem and the heat transfer characteristics have
been discussed for these models. The aim was to evalu-
ate numerical modeling capabilities to predict water va-
pour condensation from a flue gas that contains high
concentration of CO2. The results are summarized as
followings:
e to experimen-
ture. However at higher inlet temperatures
nd velocities the sensitivity to these parameters de-
oefficient was estimated by calculat-
ppreciated
an
9.
1) Both models are capable to predict the trends i
condensation process. However, the model based on
oundary layer theory shows closer valu
n
b
tal correlation. The effect of the CO2 presence in the flue
gas as a non-condensable gas was predicted correctly by
both models.
2) Heat transfer coefficient decreases as a consequence
of the increase in CO2 mass fraction for constant wall
temperature as a result of the higher resistance to diffuse
from the flue gas bulk to the boundary layer.
3) The total heat transfer rate depends on inlet velocity
and tempera
a
creases.
4) Heat transfer c
ing the interface temperature. However, it was found that
it is possible to get approximately same results by as-
suming this temperature equal to wall temperature. This
assumption facilitates the numerical efforts.
5) A brief description of the technical approach that
was implemented for current study is:
Modeling surface contact condensers with Fluent© re-
quires the Eulerian model. This Eulerian multiphase
model is an advanced model of Fluent and requires quite
a bit of experience to handle. In addition, modification of
these model to suit condensation process, which itself is
a very complex process, would require both, good under-
standing of the physical process and good knowledge of
model inside the Fluent. The accurateness of the nu-
merical modeling results is determined by the empirical
correlations specified to model the condensation process.
In the industry, there is a practice to model the process
with some correlations available in the open literature
and then tweak various parameters to results which are
close to the experimental results. Such a tuning is neces-
sary in numerical modeling as well for most of the cases,
as the general correlations may not yield accurate results
for a specific set up. It is advisable that designing a con-
denser just based on Numerical results may be a difficult
and expensive task.
8. Acknowledgements
Fluent Inc.’S solver capabilities are highly a
d I hereby knowledge use of it in the current paper.
References
[1] K. Andersson, “Combustion Tests and Modeling of the
Oxy-Fuel Process—An Overview of R&d Activities at
Chalmers University,” 2nd Oxy-Combustion Workshop at
Windsor, CT, USA, 2007.
[2] M. B. Wilkinson and J. C. Boden, “CO2 Capture via Oxy-
fuel Firing: Optimization of a Retrofit Design Concept
for a Refinery Power Station Boiler,” 1st National Con-
ference on Carbon Sequestration, Washington DC, 2001.
[3] Vattenfall, “Vattenfall’s Project on CCS—Oxyfuel
Combustion,” 2009.
http://www.vattenfall.com/en/ccs/oxyfuel-combustion.ht
m.
[4] Y. A. Cengel, “Heat Transfer: A Practical Approach,”
2nd Edition, McGraw-Hil Higher Education, Dubuque,
2003.
[5] R. K. Shah and D. P. Sekulic, “Fundamentals of Heat
Exchanger Design,” John Wiley & Sons, New Jersey,
2003. doi:10.1002/9780470172605
[6] G. F. Hewitt, “Hemisphere
Design,” Hemisphere Pu
Handbook of Heat Exchanger
blishing Corporation, Bristol,
1990.
s,”
Ph.D. Thesis, School of Engineering Sciences, Depart-
s, KTH. Stockholm, 2007.
] A. Dehbi and S. Guentay, “A Model for the Performance
-52.
[7] K. Karkoszka, “Mechanistic Modeling of Water Vapour
Condensation in Presence of Non-Condensable Gase
ment of Physic
[8
of a Vertical Tube Condenser in the Presence of
Non-Condensable Gases,” Proceeding of International
Topical Meeting on Nuclear Reactor Thermal-Hydraulics,
Vol. 177, No. 7, 1997, pp. 41
doi:10.1016/S0029-5493(97)00184-2
[9] J. M. Martín-Valdepeñas et al., “Comparison of Film
Condensation Models in Presence of Non-Condensable
Gases Implemented in a CFD Code,” Heat and Mass
Transfer, Vol. 41, No. 11, 2005, pp. 961-976.
doi:10.1007/s00231-004-0606-5
[10] J. H. Lienhard IV and J. H. Lienhard V, “A Heat Transfe
Tex
r
tbook,” 3rd Edition, Phlogiston Press, Cambridge,
hase Flow,” Springer, New York, 2006.
nd Temperature Dis-
6.2 User Guide, 2005.
2005.
[11] I. Mamoru and H. Takashi, “Thermo-Fluid Dynamics of
Two-P
[12] J. G. Collier, “Convective Boiling and Condensation,”
McGraw-Hill Co., New York, 1980.
[13] W. M. Rohsenow, “Heat Transfer a
tribution in Laminar Film Condensation,” Heat Transfer
Journal, Vol. 78, 1956, pp. 1645-1648.
[14] Fluent
[15] M. H. Kim and M. L. Corradini, “Modeling of Condensa-
tion Heat Transfer in a Reactor Containment,” Nuclear
Copyright © 2011 SciRes. EPE