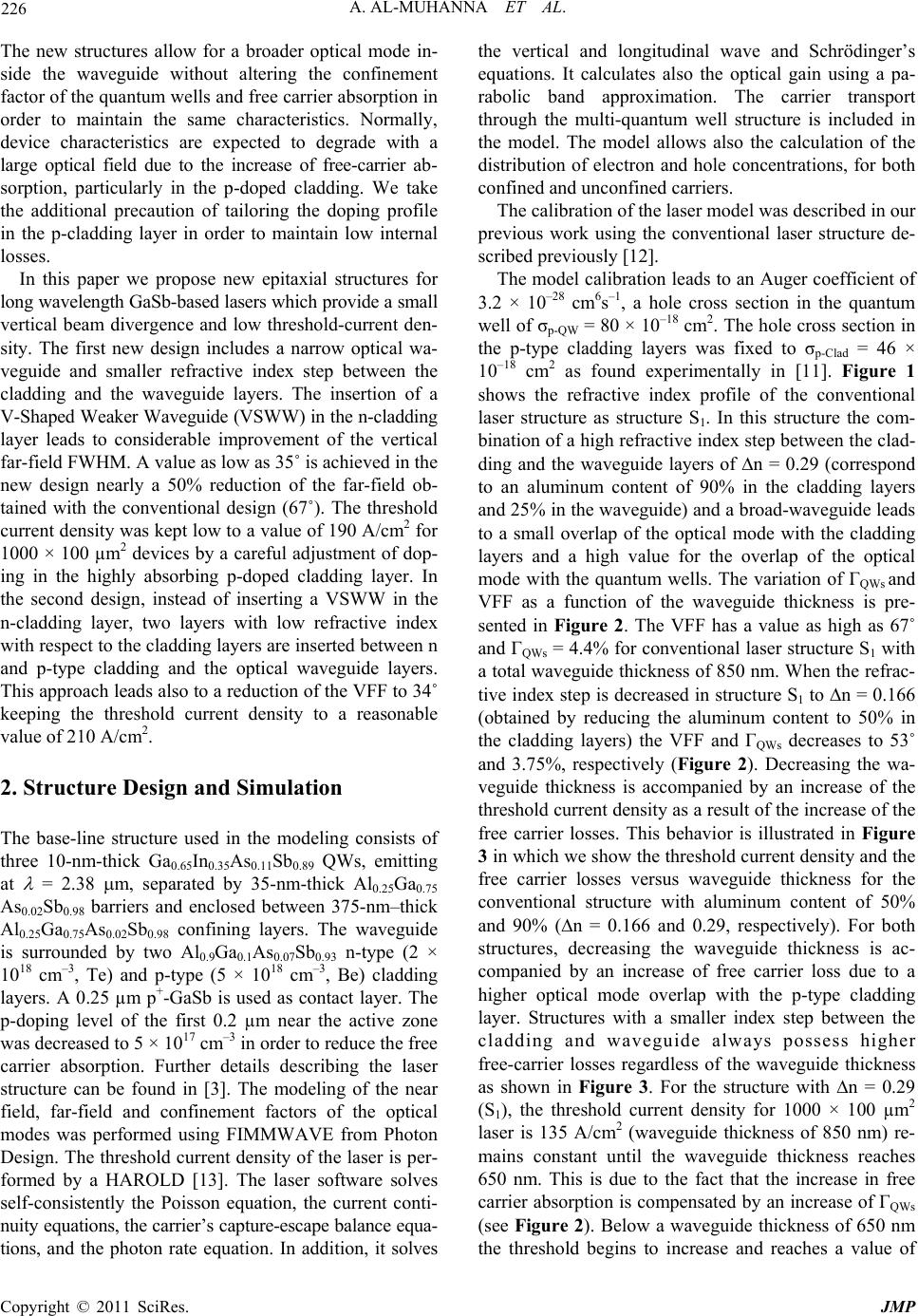
226 A. AL-MUHANNA ET AL.
The new structures allow for a broader optical mode in-
side the waveguide without altering the confinement
factor of the quantum wells and free carrier absorption in
order to maintain the same characteristics. Normally,
device characteristics are expected to degrade with a
large optical field due to the increase of free-carrier ab-
sorption, particularly in the p-doped cladding. We take
the additional precaution of tailoring the doping profile
in the p-cladding layer in order to maintain low internal
losses.
In this paper we propose new epitaxial structures for
long wavelength GaSb-based lasers which provide a small
vertical beam divergence and low threshold-current den-
sity. The first new design includes a narrow optical wa-
veguide and smaller refractive index step between the
cladding and the waveguide layers. The insertion of a
V-Shaped Weaker Waveguide (VSWW) in the n-cladding
layer leads to considerable improvement of the vertical
far-field FWHM. A value as low as 35˚ is achieved in the
new design nearly a 50% reduction of the far-field ob-
tained with the conventional design (67˚). The threshold
current density was kept low to a value of 190 A/cm2 for
1000 × 100 µm2 devices by a careful adjustment of dop-
ing in the highly absorbing p-doped cladding layer. In
the second design, instead of inserting a VSWW in the
n-cladding layer, two layers with low refractive index
with respect to the cladding layers are inserted between n
and p-type cladding and the optical waveguide layers.
This approach leads also to a reduction of the VFF to 34˚
keeping the threshold current density to a reasonable
value of 210 A/cm2.
2. Structure Design and Simulation
The base-line structure used in the modeling consists of
three 10-nm-thick Ga0.65In 0.35As0.11Sb0.89 QWs, emitting
at
= 2.38 m, separated by 35-nm-thick Al0.25Ga0.75
As0.02Sb0.98 barriers and enclosed between 375-nm–thick
Al0.25Ga0.75As0.02Sb0.98 confining layers. The waveguide
is surrounded by two Al0.9Ga0.1As0.07Sb0.93 n-type (2 ×
1018 cm–3, Te) and p-type (5 × 1018 cm–3, Be) cladding
layers. A 0.25 µm p+-GaSb is used as contact layer. The
p-doping level of the first 0.2 µm near the active zone
was decreased to 5 × 1017 cm–3 in order to reduce the free
carrier absorption. Further details describing the laser
structure can be found in [3]. The modeling of the near
field, far-field and confinement factors of the optical
modes was performed using FIMMWAVE from Photon
Design. The threshold current density of the laser is per-
formed by a HAROLD [13]. The laser software solves
self-consistently the Poisson equation, the current conti-
nuity equations, the carrier’s capture-escape balance equa-
tions, and the photon rate equation. In addition, it solves
the vertical and longitudinal wave and Schrödinger’s
equations. It calculates also the optical gain using a pa-
rabolic band approximation. The carrier transport
through the multi-quantum well structure is included in
the model. The model allows also the calculation of the
distribution of electron and hole concentrations, for both
confined and unconfined carriers.
The calibration of the laser model was described in our
previous work using the conventional laser structure de-
scribed previously [12].
The model calibration leads to an Auger coefficient of
3.2 × 10–28 cm6s–1, a hole cross section in the quantum
well of σp-QW = 80 × 10–18 cm2. The hole cross section in
the p-type cladding layers was fixed to σp-Clad = 46 ×
10–18 cm2 as found experimentally in [11]. Figure 1
shows the refractive index profile of the conventional
laser structure as structure S1. In this structure the com-
bination of a high refractive index step between the clad-
ding and the waveguide layers of ∆n = 0.29 (correspond
to an aluminum content of 90% in the cladding layers
and 25% in the waveguide) and a broad-waveguide leads
to a small overlap of the optical mode with the cladding
layers and a high value for the overlap of the optical
mode with the quantum wells. The variation of ΓQWs and
VFF as a function of the waveguide thickness is pre-
sented in Figure 2. The VFF has a value as high as 67˚
and ΓQWs = 4.4% for conventional laser structure S1 with
a total waveguide thickness of 850 nm. When the refrac-
tive index step is decreased in structure S1 to ∆n = 0.166
(obtained by reducing the aluminum content to 50% in
the cladding layers) the VFF and ΓQWs decreases to 53˚
and 3.75%, respectively (Figure 2). Decreasing the wa-
veguide thickness is accompanied by an increase of the
threshold current density as a result of the increase of the
free carrier losses. This behavior is illustrated in Figure
3 in which we show the threshold current density and the
free carrier losses versus waveguide thickness for the
conventional structure with aluminum content of 50%
and 90% (∆n = 0.166 and 0.29, respectively). For both
structures, decreasing the waveguide thickness is ac-
companied by an increase of free carrier loss due to a
higher optical mode overlap with the p-type cladding
layer. Structures with a smaller index step between the
cladding and waveguide always possess higher
free-carrier losses regardless of the waveguide thickness
as shown in Figure 3. For the structure with ∆n = 0.29
(S1), the threshold current density for 1000 × 100 µm2
laser is 135 A/cm2 (waveguide thickness of 850 nm) re-
mains constant until the waveguide thickness reaches
650 nm. This is due to the fact that the increase in free
carrier absorption is compensated by an increase of ΓQWs
(see Figure 2). Below a waveguide thickness of 650 nm
the threshold begins to increase and reaches a value of
Copyright © 2011 SciRes. JMP