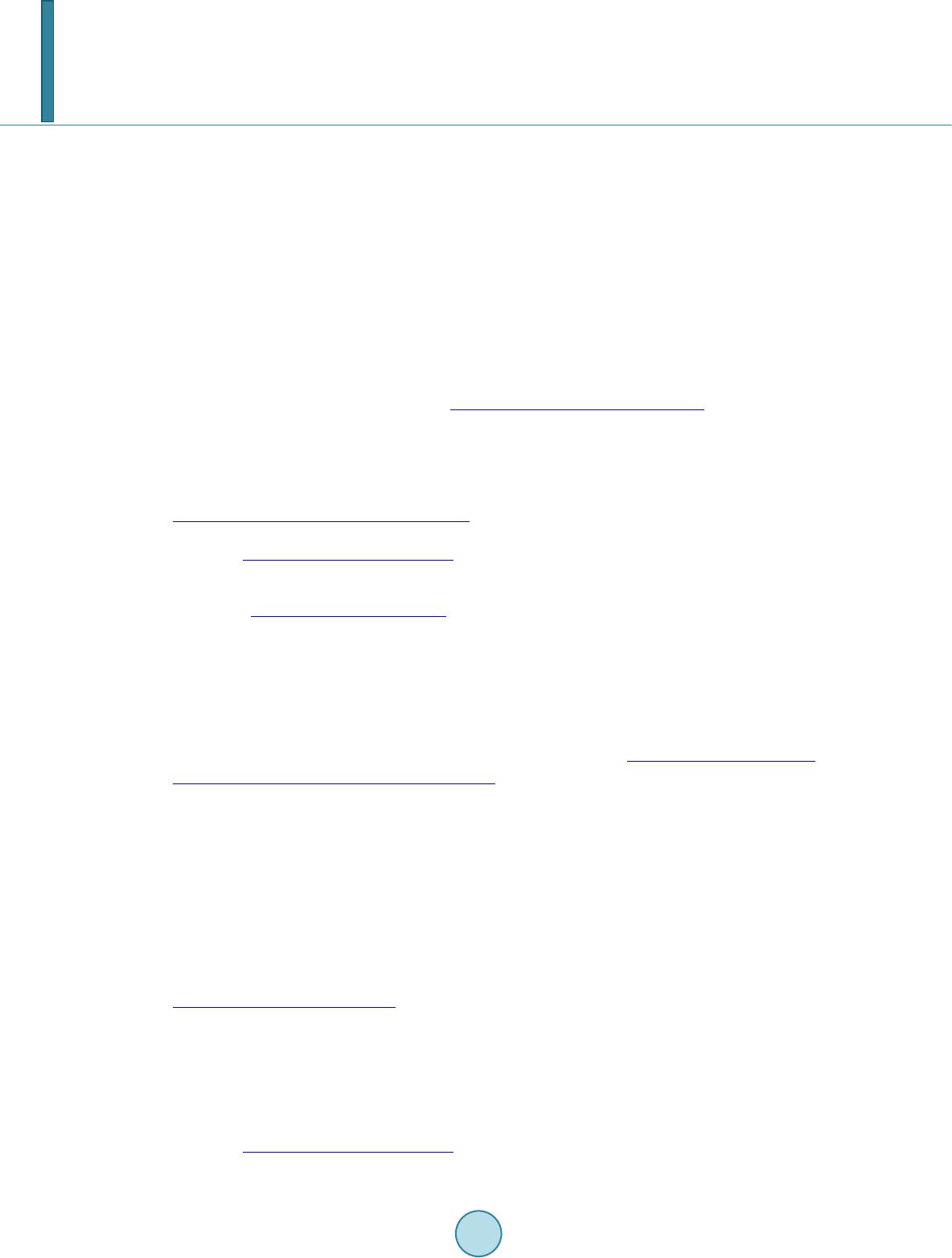
R. Le Moyne, T. Mastroianni
antimatter ramjet configur ation could be applied to a UAV propulsion system.
Design challenges, such as absorption of resultant antimatter annihilation radiation, optimization of flight
characteristics, and analysis analytical consideration of the potentially relativistic effects of the resultant anti-
matter should be addressed. For example, the restricting bounds of an ultra-intense laser incident to a gold target
generating positrons are unknown. The future improvements in the evolution of ultra-intense lasers are antic-
ipated, such as improvements in laser energy, pulse rate, and further convergence to the direct process threshold
of laser intensity. An example of the progressive evolution of chemically inherent laser propulsion is demon-
strated by Phipps, which spanned the course of three decades from theory to application. Another example of the
aerospace/aeronautical evolutionary potential can be elucidated through the evolution of flight from powered
flight to supersonic flight over the course of less than a half-century.
References
[1] Sh earer, J.W. , Garrison, J., Wong, J. and Swa i n, J.E. (1973) Pair Production by Relativistic Electrons from an Intense
Laser Focus. Physical Review A, 8, 1582-1588. http://dx.doi.org/10.1103/PhysRevA.8.1582
[2] Ch en, H., Wilks, S.C., Bonlie, J.D., Chen , S.N., Cone, K.V., Elberson, L.N., Gregori, G., Me yerhofer, D.D., Mya tt , J.,
Pri ce, D.F., Schneid er, M.B., Shepherd, R., Stafford, D.C., Tomma sin i, R., Van Maren, R. and B eiersd orfer, P. (20 09)
Making Relativistic Positrons Using Ultraintense Short Pulse Lasers. Physics of Plasmas, 16 .
[3] Ch en, H. , Wilks, S.C., Bonlie, J.D., Lia n g, E.P., Myatt, J., Pr ice, D.F., Meyerhofer, D. D. and Beiersdorfer, P. (2009)
Relativistic Positron Creation Using Ultraintense Short Pulse Lasers. Physical Review Letters, 102.
http://dx.doi.org/10.1103/PhysRevLett.102.105001
[4] Nakashi ma, K. and Takabe, H. (2002) Numerical Study of Pair Creation by Ultraintense Lasers. Physics of Plasmas, 9,
1505-1512. http://dx.doi.org/10.1063/1.1464145
[5] Phipps, C ., Birkan, M., Boh n, W., Eckel, H. A., Horisawa, H., Lippert, T., Michaelis, M., Rezun kov, Y., Sasoh , A.,
Schall , W., Sch arring, S. and Sinko, J. (2010 ) Review: Laser-Ablation Propulsion. Journal of Propulsion and Power,
26, 609-637. http://dx.doi.org/10.2514/1.43733
[6] Anderson, J.D. (1989) Introduction to Flight. McGraw-Hill, New York.
[7] Hill, P.G. and Peterso n, C.R. (19 92) Mechanics and Thermodynamics of Propulsion. Addison-Wesley, New York.
[8] Kammash, T. and Galbraith, D. (1990) Antimatter Induced Fusion Rocket Propulsio n . Proceedings of AIAA, ASM E,
SAE, and ASEE 26th Joint Propulsion Conference, AIAA 1990-2509, Orlando, Florida.
[9] Bussard , R.W. (19 60) Galactic matter and interstellar flight. Astronautica Acta, 6, 179-194.
[10] Waltrup, P.J., White, M.E., Zar l in go , F. and Gravlin, E.S. (2002) History of U.S. Navy Ramjet, Scramjet, and Mixed-
Cycle Propulsion Development. Journal of Propulsion and Power, 18, 14-27. http://dx.doi.org/10.2514/2.5928
[11] http://aerostories.free.fr/constructeurs/leduc/page8.html
[12] LeMoyne, R. (2008) Fundamental Analysis of a Combined Cycle Rocket-Ramjet Propulsion System. Proceedings of
15th AIAA International Space Planes and Hypersonic Systems and Technologies Conference, AIAA 2008-2617,
Dayton, Ohio.
[13] LeMoyne, R. (2009) Fundamental Analysis of a Tandem LH2-LOX Rocket-Ramjet Propulsion System. Proceedings of
the AIAA Space 20 09 Conference and Exposition, AIA A-2009-6658, Pasadena, California.
[14] LeMoyne, R. (2009) Fundamental Analysis of a Combined Cycle Hydrocarbon-Liquid Oxygen Tandem Ramjet-
Rocket Propulsion System. Proceedings of 16th AIAA/DLR/DGLR International Space Planes and Hypersonic Systems
and Technologies Conference, AIAA-2009-7335, Bremen.
[15] LeMoyne, R. (2011) Fundamental Analysis of a First Stage Ramjet-Rocket Combined Cycle and Second Stage Hy-
drolysis Propulsion System with Trajectory Weighting. Proceedings of 17th AIAA International Space Planes and Hy-
personic Systems and Technologies Conference AIAA-2011-2333, San Francisco.
http://dx.doi.org/10.2514/6.2011-2333
[16] Ser way, R.A. , M oses, C.J. and Moye r, C.A. (1989) Modern Physics. Saunders College, Philadelphia, Pennsylvania.
[17] Ser way, R.A. (1990) Physics for Scientists and Engineers. Saunders College, Philadelphia, Pennsylvania.
[18] Turns, S.R. (1996) An Introduction to Combustion: Concepts and Applications. McGr a w-Hill, New York.
[19] Sutton, G.P. (2006) History of Liquid Propellant Rocket Engines. AIAA, Reston, Virginia.
[20] Gah n , C., Tsakiris, G. D., P retzler, G., Witte, K.J., Thirolf, P., Ha bs, D., Delfin, C. and Wahlström, C.G. (2002) Gen-
eration of MeV Electrons and Positrons with Femtosecond Pulses from a Table -Top Laser System. Physics of Plasmas,
9, 987-999. http://dx.doi.org/10.1063/1.1446879