 Advances in Bioscience and Biotechnology, 2013, 4, 15-30 ABB http://dx.doi.org/10.4236/abb.2013.410A4003 Published Online October 2013 (http://www.scirp.org/journal/abb/) Role of TGF-β in breast cancer bone metastases Antonella Chiechi1, David L. Waning1, Keith R. Stayrook1, Jeroen T. Buijs1,2, Theresa A. Guise1, Khalid S. Mohammad1 1Division of Endocrinology, Department of Internal Medicine, Indiana University, Indianapolis, USA 2Department of Urology, Medical Center, Leiden University, Leiden, The Netherlands Email: kmohamma@iu.edu Received 29 July 2013; revised 29 August 2013; accepted 15 September 2013 Copyright © 2013 Antonella Chiechi et al. This is an open access article distributed under the Creative Commons Attribution License, which permits unrestricted use, distribution, and reproduction in any medium, provided the original work is properly cited. ABSTRACT Breast cancer is the most prevalent cancer among fe- males worldwide leading to approximately 350,000 deaths each year. It has long been known that cancers preferentially metastasize to particular organs, and bone metastases occur in ~70% of patients with ad- vanced breast cancer. Breast cancer bone metastases are predominantly osteolytic and accompanied by in- creased fracture risk, pain, nerve compression and hypercalcemia, causing severe morbidity. In the bone matrix, transforming growth factor-β (TGF-β) is one of the most abundant growth factors, which is re- leased in active form upon tumor-induced osteoclastic bone resorption. TGF-β, in turn, stimulates bone me- tastatic tumor cells to secrete factors that further drive osteolytic bone destruction adjacent to the tu- mor. Thus, TGF-β is a crucial factor responsible for driving the feed-forward vicious cycle of cancer growth in bone. Moreover, TGF-β activates epithelial-to-me- senchymal transition, increases tumor cell invasive- ness and angiogenesis and induces immunosuppres- sion. Blocking the TGF-β signaling pathway to inter- rupt this vicious cycle between breast cancer and bone offers a promising target for therapeutic intervention to decrease skeletal metastasis. This review will de- scribe the role of TGF-β in breast cancer and bone metastasis, and pre-clinical and clinical data will be evaluated for the potential use of TGF-β inhibitors in clinical practice to treat breast cancer bone metasta- ses. Keywords: Transforming Growth Factor-Beta; TGF-β; Breast Cancer; Bone Metastasis; Bone; Small Molecule Inhibitors; Antibodies; Bone Resorption 1. INTRODUCTION Cancer is the leading cause of death in economically de- veloped countries. Breast cancer is the most frequently diagnosed cancer in females accounting for 23% (1.38 million) of the total new cancer cases and is the leading cause of cancer death among females worldwide, [1,2]. It is estimated that there are nearly 3 million women living in the United States with a history of invasive breast can- cer and it is estimated that over 226,870 new cases of in- vasive breast cancer were diagnosed in 2012 [3]. Breast cancer frequently metastasizes to the skeleton, and ap- proximately 70% of patients with advanced breast will develop bone metastases [4-6]. Patients with bone metastases are at risk of skeletal complications, including spinal cord compression, pain, pathological fracture, hypercalcemia, complications due to surgery to bone, and radiation therapy. These comor- bidities are known collectively as skeletal-related events (SREs). SREs are associated with impaired mobility, re- duced quality of life, increased mortality, and higher health- care costs [7]. Standard antiresorptive treatments decrease skeletal morbidity and delay skeletal related events (SRE), but do not cause regression or cure the disease [6,8]. Cancer patients who develop bone metastases, particular- ly those with breast and prostate cancer, can survive for many years after diagnosis, during which they will suffer significant morbidity. That is why better treatments are needed to achieve the long-term goal of preventing or cu- ring bone metastases. The bone microenvironment is unique and provides fertile soil for cancers to thrive. Many growth factors and cytokines are embedded in the mineralized bone matrix and are released during osteoclastic bone resorption. Tran- sforming growth factor-β (TGF-β) is the most abundant of these factors. The TGF-β superfamily also includes other factors involved in bone homeostasis including: ac- tivins, inhibins, and bone morphogeneticproteins (BMPs). TGF-β that is released from bone is activated by either proteolytic cleavage, interaction with integrins, or pH changes in the local microenvironment [9]. In addition, OPEN ACCESS
 A. Chiechi et al. / Advances in Bioscience and Biotechnology 4 (2013) 15-30 16 TGF-β stimulates tumor production of pre-osteolytic and osteolytic factors that stimulate further bone resorption [10,11]. This categorizes TGF-β as an important factor responsible for driving the feed-forward vicious cycle of tumor growth in bone. Therefore blocking TGF-β release, its production and/or signaling is a promising strategy to treat bone metastasis. Over the past several years, several therapeutic strategies have been developed to inhibit TGF- β, including, TGF-β receptor kinase inhibitors, TGF-β neutralizing antibodies, soluble receptor decoys (Fc fu- sions) and TGF-β antisense oligonucleotides [12]. Many of these are now in early-stage clinical trials for various disease indications with particular emphasis as potential cancer therapies, including bone metastases. In this re- view, we will focus on the role of TGF-β in breast cancer and bone metastasis and discuss the potential use of no- vel TGF-β inhibiting compounds and biologics in clinical practice to treat bone metastases. 2. TGF-β STRUCTURE AND SIGNALING 2.1. TGF-β Structure TGF-β was originally named for its ability to induce ma- lignant behavior of normal fibroblasts. It is ubiquitously expressed in normal developing and adult tissues. It is a multifunctional cytokine that controls tissue homeostasis by regulating cellular processes such as apoptosis, pro- liferation and differentiation [13]. TGF-β orchestrates the response to tissue injury and mediates repair by inducing epithelial-to-mesenchymal transition (EMT) and cell mi- gration, and it is a critical regulator of the immune res- ponse. Dysregulation of TGF-β functions have been as- sociated with many disorders, including chronic fibrosis, cardiovascular diseases and cancer [14,15]. The TGF-β superfamily includes more than 30 protein ligands divided into subfamilies based on sequence simi- larity and function. Members of the TGF-β superfamily are TGF-βs, bone morphogenetic proteins (BMPs), acti- vins, inhibins, growth and differentiation factors (GDFs), NODAL and anti-Müllerian hormone (AMH) [16-18]. The ligands are all synthesized as precursors with a large N- terminal pro-domain necessary for the correct protein folding and dimerization. After cleavage, the mature li- gands form homodimers or heterodimers held together by disulfide bonds. In some cases, the prodomain is still associated with the mature protein after secretion via a non-covalent association. TGF-β is secreted as a latent precursor: After secretion the pro-domain (latency asso- ciated protein, LAP) binds and inactivate the ligand, al- lowing its association with inhibitory latent TGF binding proteins (LTBPs) that target the complex to the ECM where the latent TGF-β is sequestered. In humans, three isoforms of TGF-β have been described, TGF-β1, TGF- β2 and TGF-β3. The signaling of these three isoforms is comparable but their expression level differs across tis- sue types [19]. Signaling mediated by TGF-β ligands is transduced through cell surface recaptor complexes of two distinct types of transmembrane serine-threonine ki- nases, the type I and type II receptors. Seven type I re- ceptors (Activin-recaptor like kinases, ALKs, 1-7) and five type II receptors are known in vertebrates. The li- gand binds a type II receptor, which phosphorylates a partner type I receptor, which in turn propagates the sig- nal inside the cell via phosphorylation of downstream Smad-dependent and -independent processes [20]. 2.2. Smad-Mediated Signaling In vertebrates, eight Smad proteins are known (Smad 1- 8). Smads 1, 2, 3, 5 and 8 are the receptor-associated Smads or R-Smads. While Smad1/5/8 are phosphorylat- ed by ALK1/2/3/6 upon BMP or GDF activation, Smad2/ 3 are phosphorylated by ALK4/5/7 following TGF-β, NODAL or Activin signaling [21]. Active TGF-β binds TGF-β receptor type II (TβRII), which recruits and acti- vates ALK5. ALK5 phosphorylates R-Smad2/3, which form a heterodimeric complex with the common media- tor Smad (co-Smad or Smad4) and translocate to the nu- cleus [18,20]. Once in the nucleus, the Smad complex acts as a transcription factor able to bind chromatin and modulate its structure. To achieve a high binding affinity for the Smad-binding elements (SBE) in the TGF-β tar- get gene promoters, the Smad complex associates with other transcription factors [22,23]. Various families of transcription factors, such as forkhead, homeobox, zinc finger, AP1, Ets and basic helix-loop-helix, are Smad part- ners [23]. Moreover, the Smad complex recruits co-ac- tivators, such as p300 and CREB binding protein, or co- repressors, such as retinoblastoma-like 1 protein, to regu- late gene transcription [18,20,23]. Therefore, while Smad proteins are intrinsically transcriptional activators, the transcriptional outcome of their target genes often de- pends on the transcriptional partners associated with Smads [24]. More recently, a novel arm of TGF-β signaling has been discovered in which ALK5 activates the R-Smads, Smad1/5, leading to TGF-β-induced anchorage-indepen- dent growth and cell migration [25,26]. Furthermore, TGF-β can alternatively activate the R-Smads, Smad1/5/ 8 via the TβRI ALK1, which is mainly expressed by en- dothelial cells [27]. In fact, TGF-β/ALK1 signaling po- tentiates and TGF-β/ALK5 signaling inhibits endothelial cell proliferation and migration [28,29]. 2.3. Smad-Independent Signaling In addition to the Smad-mediated signaling, TGFβ can also activate Smad-independent signaling pathways through the interaction and association with alternative mediator Copyright © 2013 SciRes. OPEN ACCESS
 A. Chiechi et al. / Advances in Bioscience and Biotechnology 4 (2013) 15-30 17 proteins [30]. TGF-β can induce mitogen activated protein (MAP) kinase signaling, including extracellular signal regulated kinases (Erk1 and 2), p38 and c-Jun amino-terminal ki- nase (JNK) MAP kinases. The activation of Erk MAP ki- nase requires the recruitment and phosphorylation of the adaptor protein Shc, which will in turn associate with the adaptor protein Grb2 and the GTP exchange factor SOS [31]. This protein complex activates Ras to its GTP-bound form, and the kinase cascade consisting of c-Raf, MEK1 or MEK2, and Erk1 or Erk2. TGF-β also induces activa- tion of p38 and JNK MAP kinase pathway through the tumor necrosis factor (TNF) receptor-associated factor 6 (TRAF6) and TAK1. TRAF6 interacts with the TGF-β receptor complex and auto-ubiquitylates and become ac- tive. Active TRAF6 associates with TAK1, causing poly- ubiquitylation and phosphorylation of TAK1. Active TAK in turn activate p38 MAP kinase and JNK [32,33]. Furthermore, TGF-β receptor complexes interact with the polarity protein Par6 and the tight junction protein oc- cludin at epithelial cell junctions. Here, Par6 is phospho- rylated by the receptor complex, and associates with Smurf1. The Par6-Smurf1 complex confers ubiquityla- tion of RhoA and the consequent dissociation of tight junctions. The interaction of occludin with TβRI is re- quired for the localization of TbRI to tight junctions, a prerequisite for efficient TGF-β-induced dissolution of tight junctions during epithelial-mesenchymal transition [34]. Thus, the dynamic combination of canonical and non-canonical signaling cascades is responsible for the cellular responses to TGFβ signaling. 2.4. TGF-β Signaling and Epithelial-Mesenchymal Transition (EMT) TGF-β acts as a common and potent inducer of EMT via Smad-dependent and independent activation of the ex- pression of the EMT transcription factors Snail, Slug, ZEB1 and 2, and Twist [35,36]. The Smad3/4 complex directly binds the regulatory portion of the promoter of Snail, inducing its transcription. Subsequently a Smad3/ 4/Snail complex is formed that binds the regulatory pro- moter sequences of genes encoding for E-cadherin and occludin, leading to repression of their expression [37]. Smad signaling also increases the expression of ZEB transcription factors, which repress miR-200 family ex- pression, further increasing ZEB protein levels and EMT [38]. TGF-β also regulates the expression of MMP2 and 9, and ECM components (i.e. fibronectin and collagens) [39]. Moreover, TGF-β can activate EMT transcription factor expression via alternative splicing [40]. EMT is also controlled by a group of microRNAs that define changes in cytoskeleton reorganization and epi- thetlial polarity, and it is directly activated in response to TGF-β via the Smad/RhoA pathway [41]. Smad-independent TGF-β signaling pathways, such as the PI3K/Akt/mTOR pathway, result in increased protein synthesis and cell motility and invasion during EMT. TGF-β also induces EMT through ubiquitylation and su- moylation. Smad3/4 complex regulates the expression of HDM2, increasing the ubiquitylation and degradation of p53, inducing EMT progression [42]. TGF-β signaling downregulates the expression of the SUMO E3 ligase PIAS1, reducing the levels of sumoylated SnoN, and an- tagonist of TGF-β mediated EMT [43]. 3. TGF-β IN BREAST CANCER PROGRESSION TGF-β plays an essential role in maintaining homeostasis in many tissues through its ability to induce cell cycle ar- rest, differentiation and apoptosis, thereby preventing un- controlled proliferation of epithelial, endothelial and he- matopoietic cells. It is considered the most potent growth inhibitor for epithelial, hematopoietic and immune cells, because of its ability to induce cell cycle arrest, differen- tiation and apoptosis, preventing uncontrolled prolifera- tion of these cells [44,45]. However, in many cancers TGF-β signaling is compromised, because of the genetic loss of some of the pathway components or due to the downstream influence of other signaling pathways. Hence, these tumors become refractory to TGF-β growth inhibi- tion and the pro-tumorigenic actions of TGF-β may pre- vail, including immunosuppression, induction of angio- genesis and promotion of the EMT, thus facilitating can- cer migration and invasion (reviewed in [27,46,47]). 3.1. Dual Role of TGF-β in Breast Cancer Progression Transgenic mouse models have been particularly infor- mative to understand the roles of TGF-β in mammary gland development and tumor progression. Three inde- pendent studies tried to inhibit TGF-β signaling in mam- mary tissue by using the mammary gland selective mouse mammary tumor virus (MMTV) promoter to drive the expression of either a soluble TβRII:Fc fusion protein [48], a dominant negative TβRII (DNTβRII) [49] or full length TβRII antisense [50]. A proliferative mammary gland phenotype was observed in all models, consistent with the homeostatic role of TGF-β, while spontaneous mammary tumors developed only in the DNTβRII trans- genic model, but these were mostly carcinoma in situ, and arose after a prolonged latency [49]. In two additional studies, transgenic mice expressing the activated neu gene in the mammary gland were cross- ed with strains that expressed either active TGF-β1 or constitutively active TβRI/ALK5 [51,52]. A markedly delayed primary tumor development was observed in Copyright © 2013 SciRes. OPEN ACCESS
 A. Chiechi et al. / Advances in Bioscience and Biotechnology 4 (2013) 15-30 18 both cases, and tumor growth was slower than in neu single transgenic mice, underpinning a tumor suppress- ing role for TGF-β [51,52]. Nevertheless, the carcinomas that did arise in the double transgenic models were more invasive and aggressive than those occurring in MMTV- neu single transgenics. Zakharchenko et al. identified two novel TGFβ-dependent phosphorylation sites of 14- 3-3σ, Ser69 and Ser74. They found 14-3-3σ phosphory- lation to be a feed-forward mechanism in TGFβ/Smad3- dependent transcription, therefore TGFβ-dependent 14-3- 3σ phosphorylation may facilitate the formation of the protein complexes, including Smad3 and p53, at the Smad3-specific CAGA element. Also, breast tumor mouse xenograft and radiobiological assays suggested the in- volvement of phosphorylation of 14-3-3σ at Ser69 and Ser74 in the cancer progenitor population regulation and the radioresistance in breast cancer MCF7 cells. This study suggests that TGFβ-dependent phosphorylation of 14-3-3σ may play a role in the maintenance of cancer stem cells [53]. Recently, it was demonstrated that TGF- β1 down-regulated the junction adhesion molecule A (JAM-A) expression via its effects on both the transcrip- tional and post-translational regulations of JAM-A thus attenuating cell adhesion and promoting cell invasion and that the effect of TGF-β was achieved via the activa- tion of Smads [54]. Also, a recent study identifies miR- 155-mediated loss of C/EBPβ as the mechanism that shifts TGF-β response in breast cancer from growth inhi- bition to EMT, invasion and metastasis, promoting breast cancer progression. C/EBPβ seems to work as a tran- scriptional activator of genes encoding the epithelial junction proteins E-cadherin and coxsackie virus and adenovirus receptor [55]. These and other [56,57] studies have provided strong support for a tumor-suppressive role for epithelial TGF-β signaling in mammary gland tu- morigenesis. However, while TGF-β may inhibit the growth of mammary tumors in the early stages, it also appears, in these models, to enhance the metastatic po- tential of those carcinomas that are able to overcome the TGF-β-dependent growth suppression and develop. 3.2. TGF-β Expression Levels in Human Breast Cancer When the TGF-β suppressive effects are lost, TGF-β overproduction is commonly observed in many solid tumors. TGF-β expression level is often higher in breast cancer compared to normal mammary gland tissue and it appears to increase in the advanced stages of tumor pro- gression [58-60]. Moreover, TGF-β expression levels correlate with prognosis and angiogenesis in breast can- cer patients [61]. Plasma TGF-β1 expression has also been found increased in breast cancer patients, and its level correlates with disease stage [62-65]. Plasma TGF- β1 levels have a prognostic value also after tumor resec- tion: patients whose plasma TGF-β1 levels normalized after surgery had a better prognosis than those patients with persistently elevated levels, who had higher risk of lymph node metastases and disease progression [64]. These data may suggest an important causal role for TGF-β in metastases and disease progression. Plasma TGF-β1 levels have also been determined in 49 bone metastasis patients, including 23 breast cancer patients, and were reported to be elevated in more than half of the cancer patients and positively correlated with TGF-β signaling related markers, including parathyroid thyroid hormone-related peptide (PTHrP) and interleukin 10(IL-10) [66]. A recent study shows that elevated circu- lating levels of TGF-β and CXCL1 are associated with a poor prognosis, and higher detection of circulating tumor cells and propensity of these cells to seed lung metasta- ses in patients with breast cancer [67]. TGF-β plasma levels may be indicative of TGF-β-de- pendent metastatic disease and may be useful biomarkers to predict the success of treatment with TGF-β antago- nists in metastatic disease. Ongoing clinical trials are trying to answer these questions. In addition, a highly sig- nificant association between TβRII expression and re- duced survival has been detected in patients bearing es- trogen receptor negative breast cancer [68]. Richardsen et al. recently published immunohistochemical data from 38 cancer patients: high TGF-β levels can be detected in both primary and metastatic tumors and high stromal TGF-β expression is associated with increased mortality [69]. Due to TGF-β dual nature in breast cancer, its use as single tumor marker that might distinguish patients with high risk of metastases is unlikely. Molecules involved in the TGF-β downstream signaling play an important role in determining TGF-β prognostic implication, as shown in a retrospective cohort study in patients with invasive non-metastatic breast cancer. High expression of Smad4 showed a trend for better prognosis while high expres- sion of pphosphorylated-Smad2 was associated with poor prognosis [70]. 3.3. TGF-β and Breast Cancer Ste m Cells An increasing body of basic and clinical studies have provided evidence of self-renewing, stem/progenitor-like cells within solid tumors, which have also been referred to as cancer stem cells (CSCs) [71-77]. CSCs are beliv- ed to constitute a small minority of neoplastic cells with- in a given tumor and are defined by their ability to pro- pagate a tumor and potentially seed new metastases [74]. The concept of CSCs remarks the importance of target- ing the correct cell population in cancer therapy in order to obtain better results in terms of survival and tumor re- lapse. Conventional treatments aim to eliminate the rap- idly dividing cells in a tumor, leaving space and time to Copyright © 2013 SciRes. OPEN ACCESS
 A. Chiechi et al. / Advances in Bioscience and Biotechnology 4 (2013) 15-30 19 the slower proliferating, less differentiated CSCs to re- populate the tumor [78]. By sorting breast cancer cells for a normal mammary stem cell phenotype (CD44+/CD24−/low), Al-Hajj et al. was the first to isolate the breast CSC fraction [71]. More recently, Shipitsin et al. demonstrated that vimentin, con- nective tissue growth factor (CTGF), PAI-1, osteonectin, as well as TβRII were coexpressed with CD44 [79]. In fact, many of the genes actively transcribed by CD44+ cells were associated with a mesenchymal phenotype and many were known TGF-β target genes. They were also able to associate this gene signature with poor prognosis [79]. Mani et al. demonstrated that EMT generates cells with properties of stem cells [80]. TGF-β-induced EMT in immortalized human mammary epithetlial cells (HMEC) was associated with the acquisition of the CD44+/ CD24−/low phenotype and mesenchymal traits, and in- creased ability to form mammospheres, a property asso- ciated with mammary epithelial stem cells [81]. Para- crine and autocrine signals induce and maintain mesen- chymal and stem cell states in the breast [81]. In addition, forcing EMT by overexpressing the EMT transcription factors (and TGF-β target genes) SNAIL1 or TWIST also resulted in a CD44+/CD24−/low phenotype that displayed enhanced tumorigenic potential when injected in mice. In a recent study, the loss of DUSP4, a downstream mole- cule in the TGF-β apoptosis signaling pathway, was shown to increase mammosphere formation and the expression of the CSC-promoting cytokines IL-6 and IL-8 in a mo- del of basal-like breast cancer (BLBC). These effects were caused in part by loss of control of the MEK and JNK pathways and involved downstream activation of the ETS-1 and c-JUN transcription factors. Enforced ex- pression of DUSP4 reduced the CD44+/CD24− popula- tion in multiple BLBC cell lines in a MEK-dependent manner, limiting tumor formation, again underpinning the dual role of TGF-β in breast cancer [82]. Taken together, these studies provide evidence that TGF-β is important in regulating the dynamics of cancer cell populations by favoring CSC selfrenewal and inhibiting the commitment to differentiation. 4. TGF-Β, BREAST CANCER AND BONE METASTASIS 4.1. Normal Bone Physiology Bone is primarily made of type I collagen that is miner- alized by hydroxyapatite. By weight, bone is about 60% mineral, 10% water and 30% organic matrix. Mineral are made of hydroxyapatite crystals a naturally occurring calcium phosphate. The organic matrix is 98% type I col- lagen and 2% noncollagenous protein. The noncollage- nous proteins include growth factors and cytokines, and extracellular matrix proteins such as osteonectin, osteo- pontin, bone sialoprotein, osteocalcin and proteoglycans. Although noncollangenous components make small con- tributions to the overall bone volume it represents major contributions to its biologic function. Growth factors and cytokines such as transforming growth factor-β (TGF-β), bone morphogenetic proteins (BMPs) osteoprotegerin (OPG), insulin-like growth factor (IGF), interferon-γ, the tumor necrosis factors (TNFs) and the interleukins (ILs), are present in very small quantities in bone matrix but have critical effects regulating bone cell differentiation, activation and growth. The cellular component of bone that is associated with the bone homeostasis the bone resorbing osteoclasts, the bone forming osteoblasts and the cell embedded in the bone matrix, the osteocytes. Osteoclasts arise from he- matopoietic progenitors that also give rise to mono- cyte/macrophages lineage. The osteoclast precursor cells are recruited to the bone surface where they fuse to form large multinucleated cell. The interaction of the osteo- clast precursors with the stromal cell and the osteoblasts, in the presence of several intermediary factors such as PTH, Vitamin-D , IL-6, IL-11 and PGE2, induces the central mediator of osteoclast differentiation the RANKL. RANKL or receptor activator of NFκB is a membrane bound member of the TNF receptor family expressed at the osteoblast surface. RANKL binds to its receptor, RANK, which is expressed on the surface of osteoclast precursor. The interaction of the RANKL with its recap- tor RANK stimulates the osteoclast differentiation. RANKL/RANK knockout mice develop severe osteo- petrosis due to total lack of osteoclast [83,84]. OPG is another TNF superfamliy member, it a soluble factor that act as a decoy receptor for RANKL. When RANKL binds to OPG it prevents the interaction of RANKL with RANK and inhibits osteoclast activation. OPG knockout mice develop osteoporosis as a result of increase in number of osteoclast. The bone forming osteoblasts are derived from mes- enchymal stem cells, pluripotent cells that can differenti- ate into a variety of cell types including myoblasts, adi- pocytes, chondrocytes, osteoblasts, and osteocytes. Osteoblasts are the bone cells that secrete the organic matrix within which several growth factors are embed- ded. Runx2 and Osterix are two transcription factors that are required for osteoblast formation and differentiation. The regulatory activity of these central osteoblast regu- lators is modified by cofactors including members of the Dlx (distaless), Msx, and Hox homeodomain gene fami- lies and downstream signal transduction mediators such as the TGF-β superfamily-related SMADs. As active os- teoblasts produce bone matrix (osteoid), they become embedded into their own product and at this stage it is called an osteocyte. Osteocytes make up to 95% of all bone cells. Osteo- Copyright © 2013 SciRes. OPEN ACCESS
 A. Chiechi et al. / Advances in Bioscience and Biotechnology 4 (2013) 15-30 20 cytes create an interconnected network in bone allowing for intercellular communications between each other and the surface-lining osteoblasts [85]. Osteocyte senses me- chanical load through their canalicular processes and ini- tiate a series of biochemical signaling events that coor- dinate and influence the activity of osteoprogenitor cell, osteoblasts and osteoclasts, which in turn respond by remodeling bone mass [86,87]. Sclerostin, a secreted pro- tein expressed by osteocytes, responds to mechanical load. Sclerostin plays a central role in the anabolic response of bone to mechanical. Mechanical loads repress Sclerostin mRNA and protein expression thus, releasing the inhibi- tion on new bone synthesis [88]. Bone is a dynamic structure and adult bone is con- tinuously remodeled by the coordinated activities of bone- resorbing osteoclasts and bone-forming osteoblasts [89]. The continuous remodeling process is necessary to re- place defective bone as well as to release calcium for va- rious metabolic processes It is the balance between the osteoclasts and osteoblast activity is what keep constant bone mass and the disruption of this balance will result is significant pathological condition such as osteoporosis or osteopetrosis. 4.2. TGF-β and Bone Interaction The involvement of growth factors, cytokines and cell adhesion molecules in the remodeling process is what makes bone an attractive site for cancer metastases. TGF-β1 is one of the most abundant growth factors in bone matrix [90]. It is an essential factor for bone re- modeling and can affect both bone formation and resorp- tion. The effects of TGF-β on osteoblast, osteoclasts and bone remodeling are complex and are both spatial and temporal-dependent [91]. Bone is resorbed by osteoclasts and when the resorption process is completed, a reversal period follows after which osteoblasts deposit new bone matrix to fill the resorption cavity, a process known as coupling. The newly deposited collagenous matrix will be mineralized following a resting phase. Evidence is accumulating that TGF-β is a key media- tor in coupling bone resorption to bone formation [92]. Osteoblasts secrete TGF-β, where it is embedded into the mineralized bone matrix [93,94]. TGF-β is stored in the bone matrix in a latent form. Upon bone resorption by osteoclasts, TGF-β is release and activated which in turn activates the proliferation of osteoblast precursor which migrates to the sites of bone resorption [95]. The exposed bone mineral matrix and release of osteotropic factors, such as bone morphogenetic proteins (BMPs), insulin growth factor (IGF)-I and -II, and platelet derived growth factor (PDGF), may then promote differentiation of the osteoblast precursor to osteoblasts [96]. It was shown that TGF-β block osteoblast differentiation and bone mi- neralization in later phases of osteoblastic differentiation [97]. In a coculture of osteoclast precursors with osteo- blast and stromal cells, TGF-β was shown to inhibit re- sorption factors such as RANKL and M-CSF while acti- vating the expression of osteoclast inhibitors such as OPG [98,99]. TGF-β is a major regulator of osteoclast function ei- ther directly or indirectly through its effect on osteoblast. The importance of TGF-β on osteoclastogenesis is clear but the exact mechanism is unclear. During bone resorp- tion osteoclasts secrete cathepsins, which proteolytically release activate TGF-β from the latent complex [100,101] and because osteoclast express both TGF-β and its re- ceptors they can respond directly to TGF-β signaling. TGF-β can inhibit the recruitment of osteoclast precur- sors in fetal bone culture but enhances bone resorption by stimulating proliferation and differentiation of osteo- clast precursors. TGF-β also enhances osteoblast lineage RANKL expression, thus promoting osteoclast precursor recruitment [102]. It has been recently reported by Nguyen, et al., that mechanical load rapidly represses the net activity of the TGF-β pathway in osteocytes. This result in reduced phosphorylation and activity Smad2 and Smad3 thus com- promises the anabolic response of bone to mechanical load, demonstrating that the mechanosensitive regulation of TGF-β signaling is essential for load-induced bone for- mation [103]. 4.3. TGF-β and Osteolityc Bone Metastases Bone is a common site of dissemination for breast cancer. The bone microenvironment consists of a rich store of multiple growth factors including TGF-β. The metaphy- seal bone, which is predominantly composed of trabecu- lar bone and is highly vascular, appears to be the prefer- red site for bone metastases. Bone metastases develop in about 70% of patients with advanced breast cancer. This is usually a late complication of cancer that can lead to debilitating skeletal related events such as pain, fractures, hypercalcemia and nerve compression which reduce the patient’s quality of life [5,6]. Metastasis to bone is a complete multistep process of events that involves an interaction between the tumor and the host cells. This multifaceted process consists of a series of steps whereby cancer cells detach from the pri- mary tumor, enter into the circulation, disseminate to dis- tal bone sinusoids, enter the bone marrow by extravasa- tion, adapt to the new microenvironment, and eventually grow into lethal tumor which colonies the bone [104]. In bone metastasis biopsies from patients with breast cancer, 75% show positive nuclear staining for phospho- rylated-Smad2, as seen on histological sections, indicat- ing an active TGF-β signaling [105]. It has been well established in the literature that TGF- Copyright © 2013 SciRes. OPEN ACCESS
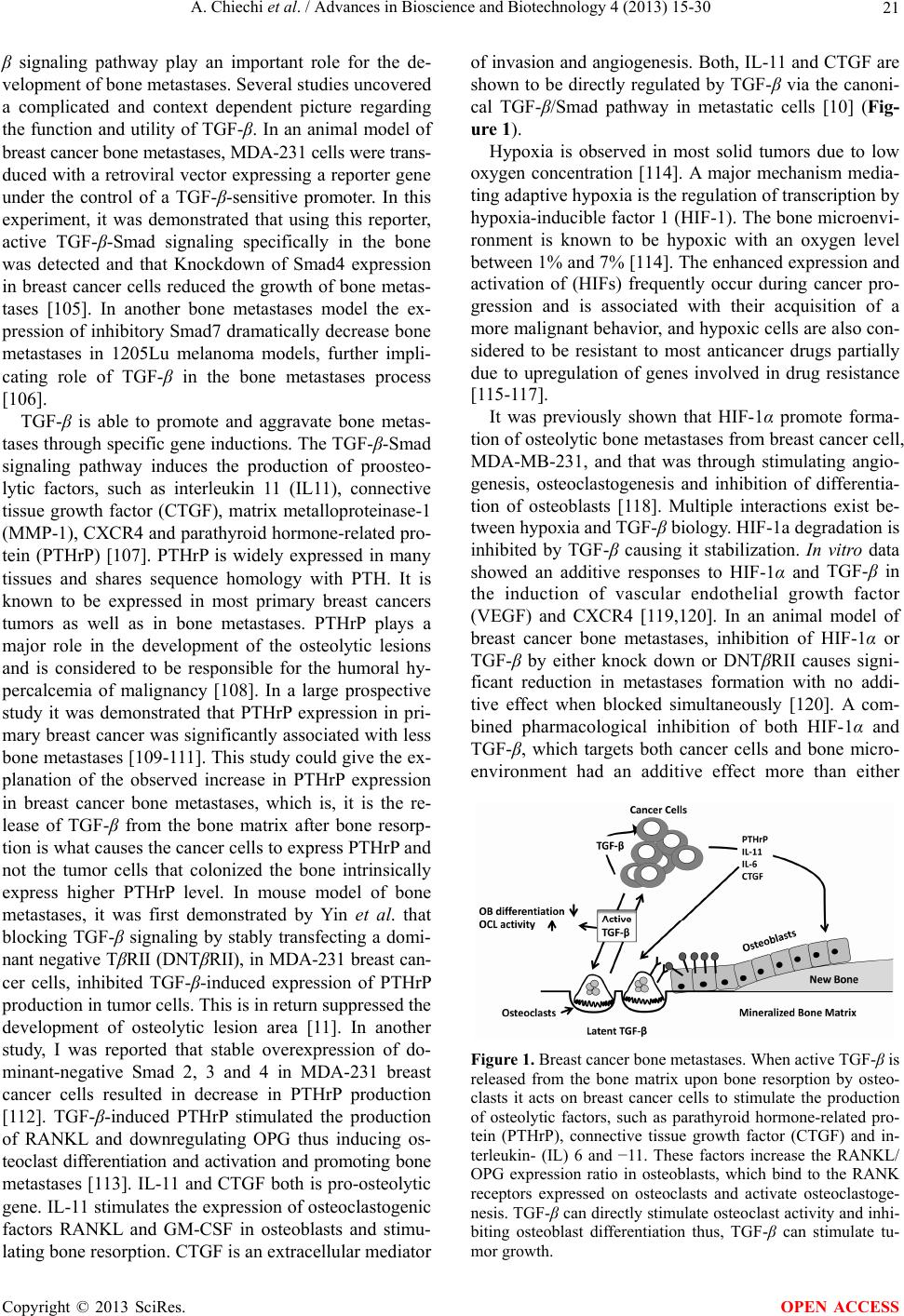 A. Chiechi et al. / Advances in Bioscience and Biotechnology 4 (2013) 15-30 21 β signaling pathway play an important role for the de- velopment of bone metastases. Several studies uncovered a complicated and context dependent picture regarding the function and utility of TGF-β. In an animal model of breast cancer bone metastases, MDA-231 cells were trans- duced with a retroviral vector expressing a reporter gene under the control of a TGF-β-sensitive promoter. In this experiment, it was demonstrated that using this reporter, active TGF-β-Smad signaling specifically in the bone was detected and that Knockdown of Smad4 expression in breast cancer cells reduced the growth of bone metas- tases [105]. In another bone metastases model the ex- pression of inhibitory Smad7 dramatically decrease bone metastases in 1205Lu melanoma models, further impli- cating role of TGF-β in the bone metastases process [106]. TGF-β is able to promote and aggravate bone metas- tases through specific gene inductions. The TGF-β-Smad signaling pathway induces the production of proosteo- lytic factors, such as interleukin 11 (IL11), connective tissue growth factor (CTGF), matrix metalloproteinase-1 (MMP-1), CXCR4 and parathyroid hormone-related pro- tein (PTHrP) [107]. PTHrP is widely expressed in many tissues and shares sequence homology with PTH. It is known to be expressed in most primary breast cancers tumors as well as in bone metastases. PTHrP plays a major role in the development of the osteolytic lesions and is considered to be responsible for the humoral hy- percalcemia of malignancy [108]. In a large prospective study it was demonstrated that PTHrP expression in pri- mary breast cancer was significantly associated with less bone metastases [109-111]. This study could give the ex- planation of the observed increase in PTHrP expression in breast cancer bone metastases, which is, it is the re- lease of TGF-β from the bone matrix after bone resorp- tion is what causes the cancer cells to express PTHrP and not the tumor cells that colonized the bone intrinsically express higher PTHrP level. In mouse model of bone metastases, it was first demonstrated by Yin et al. that blocking TGF-β signaling by stably transfecting a domi- nant negative TβRII (DNTβRII), in MDA-231 breast can- cer cells, inhibited TGF-β-induced expression of PTHrP production in tumor cells. This is in return suppressed the development of osteolytic lesion area [11]. In another study, I was reported that stable overexpression of do- minant-negative Smad 2, 3 and 4 in MDA-231 breast cancer cells resulted in decrease in PTHrP production [112]. TGF-β-induced PTHrP stimulated the production of RANKL and downregulating OPG thus inducing os- teoclast differentiation and activation and promoting bone metastases [113]. IL-11 and CTGF both is pro-osteolytic gene. IL-11 stimulates the expression of osteoclastogenic factors RANKL and GM-CSF in osteoblasts and stimu- lating bone resorption. CTGF is an extracellular mediator of invasion and angiogenesis. Both, IL-11 and CTGF are shown to be directly regulated by TGF-β via the canoni- cal TGF-β/Smad pathway in metastatic cells [10] (Fig- ure 1). Hypoxia is observed in most solid tumors due to low oxygen concentration [114]. A major mechanism media- ting adaptive hypoxia is the regulation of transcription by hypoxia-inducible factor 1 (HIF-1). The bone microenvi- ronment is known to be hypoxic with an oxygen level between 1% and 7% [114]. The enhanced expression and activation of (HIFs) frequently occur during cancer pro- gression and is associated with their acquisition of a more malignant behavior, and hypoxic cells are also con- sidered to be resistant to most anticancer drugs partially due to upregulation of genes involved in drug resistance [115-117]. It was previously shown that HIF-1α promote forma- tion of osteolytic bone metastases from breast cancer cell, MDA-MB-231, and that was through stimulating angio- genesis, osteoclastogenesis and inhibition of differentia- tion of osteoblasts [118]. Multiple interactions exist be- tween hypoxia and TGF-β biology. HIF-1a degradation is inhibited by TGF-β causing it stabilization. In vitro data showed an additive responses to HIF-1α and TGF- β in the induction of vascular endothelial growth factor (VEGF) and CXCR4 [119,120]. In an animal model of breast cancer bone metastases, inhibition of HIF-1α or TGF-β by either knock down or DNTβRII causes signi- ficant reduction in metastases formation with no addi- tive effect when blocked simultaneously [120]. A com- bined pharmacological inhibition of both HIF-1α and TGF-β, which targets both cancer cells and bone micro- environment had an additive effect more than either Figure 1. Breast cancer bone metastases. When active TGF-β is released from the bone matrix upon bone resorption by osteo- clasts it acts on breast cancer cells to stimulate the production of osteolytic factors, such as parathyroid hormone-related pro- tein (PTHrP), connective tissue growth factor (CTGF) and in- terleukin- (IL) 6 and −11. These factors increase the RANKL/ OPG expression ratio in osteoblasts, which bind to the RANK receptors expressed on osteoclasts and activate osteoclastoge- nesis. TGF-β can directly stimulate osteoclast activity and inhi- biting osteoblast differentiation thus, TGF-β can stimulate tu- mor growth. Copyright © 2013 SciRes. OPEN ACCESS
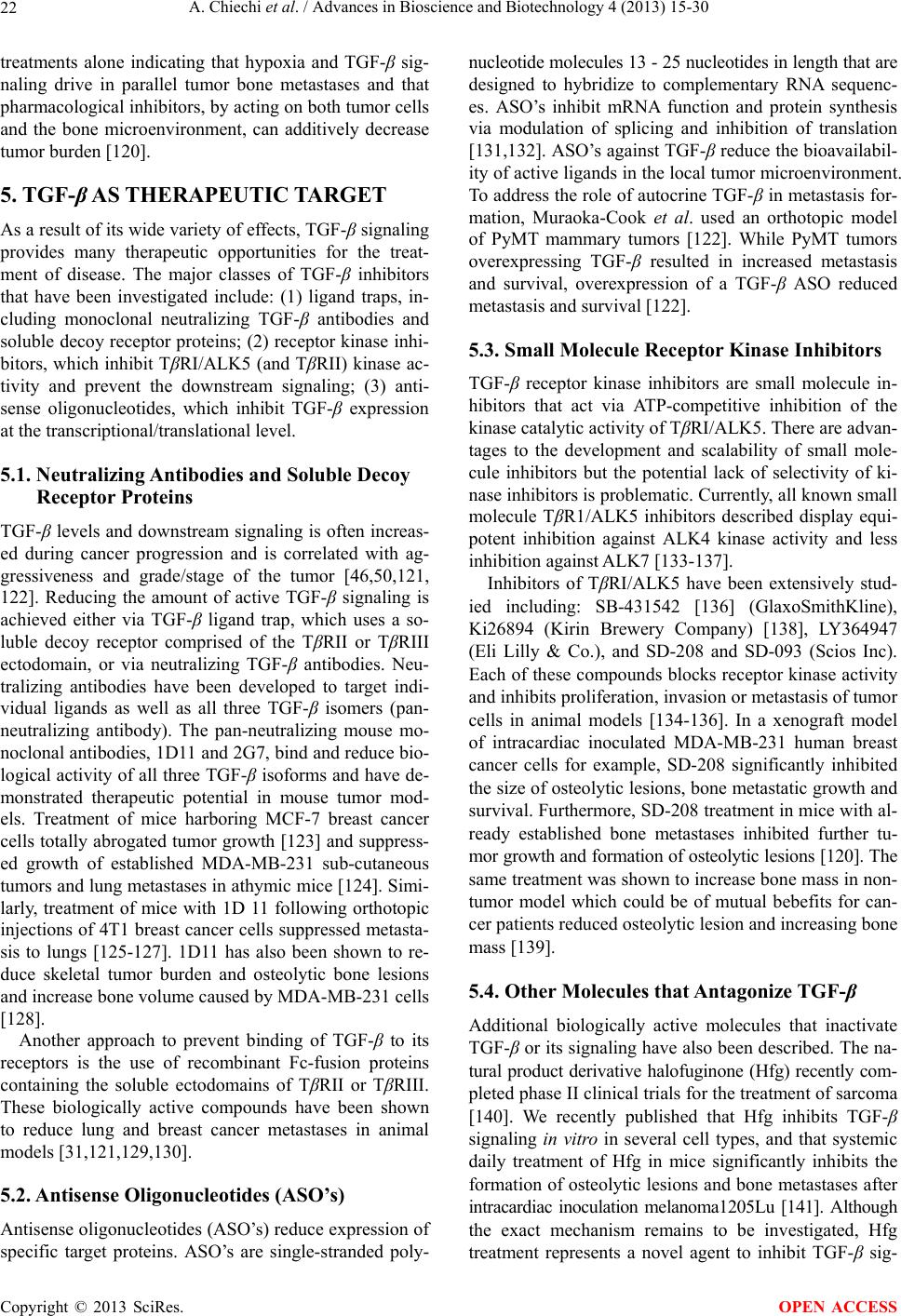 A. Chiechi et al. / Advances in Bioscience and Biotechnology 4 (2013) 15-30 22 treatments alone indicating that hypoxia and TGF-β sig- naling drive in parallel tumor bone metastases and that pharmacological inhibitors, by acting on both tumor cells and the bone microenvironment, can additively decrease tumor burden [120]. 5. TGF-β AS THERAPEUTIC TARGET As a result of its wide variety of effects, TGF-β signaling provides many therapeutic opportunities for the treat- ment of disease. The major classes of TGF-β inhibitors that have been investigated include: (1) ligand traps, in- cluding monoclonal neutralizing TGF-β antibodies and soluble decoy receptor proteins; (2) receptor kinase inhi- bitors, which inhibit TβRI/ALK5 (and TβRII) kinase ac- tivity and prevent the downstream signaling; (3) anti- sense oligonucleotides, which inhibit TGF-β expression at the transcriptional/translational level. 5.1. Neutralizing Antibodies and Soluble Decoy Receptor Proteins TGF-β levels and downstream signaling is often increas- ed during cancer progression and is correlated with ag- gressiveness and grade/stage of the tumor [46,50,121, 122]. Reducing the amount of active TGF-β signaling is achieved either via TGF-β ligand trap, which uses a so- luble decoy receptor comprised of the TβRII or TβRIII ectodomain, or via neutralizing TGF-β antibodies. Neu- tralizing antibodies have been developed to target indi- vidual ligands as well as all three TGF-β isomers (pan- neutralizing antibody). The pan-neutralizing mouse mo- noclonal antibodies, 1D11 and 2G7, bind and reduce bio- logical activity of all three TGF-β isoforms and have de- monstrated therapeutic potential in mouse tumor mod- els. Treatment of mice harboring MCF-7 breast cancer cells totally abrogated tumor growth [123] and suppress- ed growth of established MDA-MB-231 sub-cutaneous tumors and lung metastases in athymic mice [124]. Simi- larly, treatment of mice with 1D 11 following orthotopic injections of 4T1 breast cancer cells suppressed metasta- sis to lungs [125-127]. 1D11 has also been shown to re- duce skeletal tumor burden and osteolytic bone lesions and increase bone volume caused by MDA-MB-231 cells [128]. Another approach to prevent binding of TGF-β to its receptors is the use of recombinant Fc-fusion proteins containing the soluble ectodomains of TβRII or TβRIII. These biologically active compounds have been shown to reduce lung and breast cancer metastases in animal models [31,121,129,130]. 5.2. Antisense Oligonucleotides (ASO’s) Antisense oligonucleotides (ASO’s) reduce expression of specific target proteins. ASO’s are single-stranded poly- nucleotide molecules 13 - 25 nucleotides in length that are designed to hybridize to complementary RNA sequenc- es. ASO’s inhibit mRNA function and protein synthesis via modulation of splicing and inhibition of translation [131,132]. ASO’s against TGF-β reduce the bioavailabil- ity of active ligands in the local tumor microenvironment. To address the role of autocrine TGF-β in metastasis for- mation, Muraoka-Cook et al. used an orthotopic model of PyMT mammary tumors [122]. While PyMT tumors overexpressing TGF-β resulted in increased metastasis and survival, overexpression of a TGF-β ASO reduced metastasis and survival [122]. 5.3. Small Molecule Receptor Kinase Inhibitors TGF-β receptor kinase inhibitors are small molecule in- hibitors that act via ATP-competitive inhibition of the kinase catalytic activity of TβRI/ALK5. There are advan- tages to the development and scalability of small mole- cule inhibitors but the potential lack of selectivity of ki- nase inhibitors is problematic. Currently, all known small molecule TβR1/ALK5 inhibitors described display equi- potent inhibition against ALK4 kinase activity and less inhibition against ALK7 [133-137]. Inhibitors of TβRI/ALK5 have been extensively stud- ied including: SB-431542 [136] (GlaxoSmithKline), Ki26894 (Kirin Brewery Company) [138], LY364947 (Eli Lilly & Co.), and SD-208 and SD-093 (Scios Inc). Each of these compounds blocks receptor kinase activity and inhibits proliferation, invasion or metastasis of tumor cells in animal models [134-136]. In a xenograft model of intracardiac inoculated MDA-MB-231 human breast cancer cells for example, SD-208 significantly inhibited the size of osteolytic lesions, bone metastatic growth and survival. Furthermore, SD-208 treatment in mice with al- ready established bone metastases inhibited further tu- mor growth and formation of osteolytic lesions [120]. The same treatment was shown to increase bone mass in non- tumor model which could be of mutual bebefits for can- cer patients reduced osteolytic lesion and increasing bone mass [139]. 5.4. Other Molecules that Antagonize TGF-β Additional biologically active molecules that inactivate TGF-β or its signaling have also been described. The na- tural product derivative halofuginone (Hfg) recently com- pleted phase II clinical trials for the treatment of sarcoma [140]. We recently published that Hfg inhibits TGF-β signaling in vitro in several cell types, and that systemic daily treatment of Hfg in mice significantly inhibits the formation of osteolytic lesions and bone metastases after intracardiac inoculation melanoma1205Lu [141]. Although the exact mechanism remains to be investigated, Hfg treatment represents a novel agent to inhibit TGF-β sig- Copyright © 2013 SciRes. OPEN ACCESS
 A. Chiechi et al. / Advances in Bioscience and Biotechnology 4 (2013) 15-30 23 naling in bone metastasis. 5.5. Combination Therapy An attractive approach to increase treatment efficacy for patients with bone metastases is to combine treatments that antagonize the effects of TGF-β with other therapies. For example, targeting TGF-β signaling can enhance the therapeutic efficacy of various cytotoxic agents as was recently shown for rapamycin [142] and doxorubicin [143,144]. Studies in our laboratory show that SD-208 dosed in combination with an inhibitor of bone resorp- tion, zoledronic acid, reduces the progression of establi- shed osteolytic metastases from breast cancer more ef- fectively than either therapy alone [145]. Using the same bone metastasis model of intracardiac inoculation of MDA- MB-231 breast cancer cells, we tested the effects of a combined treatment of SD-208 and 2-methoxyestradiol, an inhibitor of HIF-1α, the key mediator of hypoxia. Com- bined treatment with these agents reduces osteolytic le- sions, tumor burden and improves survival of mice more effectively than either treatment alone [120]. 5.6. Risks, Limitations and Opportunities As a result of its biological importance and wide variety of effect, blockade of TGF-β or its signaling provides in- triguing therapeutic opportunities for the treatment of many different disease indications. However, potent and/ or chronic inhibition of this wide-spread biologically im- portant molecule may also potentially result in a variety of undesirable side effects. Drug delivery challenges must be overcome for ASO- based therapies and large biological (neutralizing anti- bodies). The generation of small molecules such as TGF- β receptor kinase inhibitors overcomes the necessity of injectable delivery, loss of efficacy due to neutralizing antibody generation and/or tissue penetration issues com- monly observed with biologic-based agents and most are suitable for oral dosing [12]. However, TGF-β recaptor ki- nase inhibitors used so far are less selective than the cur- rent TGF-β ASO’s or biologic-based TGF-β-directed the- rapies. Other promising approaches to overcome off-target tissue toxicity and poor drug exposure to tumor cells in bone metastatic disease are the use of bisphosphonate to deliver therapeutics directly to bone. One approach is a bisphosphonate-coated liposome, which may be useful as a targeting device to sites of high bone turnover, include- ing sites with bone metastatic disease [146]. Another pos- sibility is targeting anti-TGF-β therapies via conjugation of small molecule inhibitors to bisphosphonates. Poten- tially, these bone-targeted strategies may allow for a more prolonged local exposure to higher concentrations of the compounds thereby enhancing therapeutic efficacy and minimizing systemic side effects. Additionally, these bi- oactive compounds of interest could be delivered to bone metastatic sites in combination with other anticancer agents with synergistic or mechanistic action. 6. CONCLUSION TGF-β is a pluripotent cytokine with a prominent role in breast cancer progression and bone metastasis. TGF-β is a central mediator in driving a feed-forward vicious cy- cle of tumor growth in bone. Thus much effort has been placed on development of agents to inhibit TGF-β activi- ity. Currently, three therapeutic modalities targeting TGF- β have been pursued and are presently being tested in clinical trials in cancer patients (incl. bone metastatses): TGF-β antibodies, TGF-β receptor kinase inhibitors and TGF-β antisense oligonucleotides. TGF-β has many other functions in normal physiology, and may also act as a tumor suppressor in certain malignancies. Therefore con- cerns will remain that long-term blockade of this path- way may have other off-target effects. The next decade should reveal new and exciting clinical data that will help determine which TGF-β therapeutic strategies are most effective for the treatment of patients. REFERENCES [1] Jemal, A., Bray, F., Center, M.M., Ferlay, J., Ward, E. and Forman, D. (2011) Global cancer statistics. CA: A Cancer Journal for Clinicians, 61, 69-90. http://dx.doi.org/10.3322/caac.20107 [2] Siegel, R., DeSantis, C., Virgo, K., Stein, K., Mariotto, A., Smith, T., Cooper, D., Gansler, T., Lerro, C., Fedewa, S., Lin, C., Leach C, Cannady, R.S., Cho, H., Scoppa, S., Hachey, M., Kirch, R., Jemal, A. and Ward, E. (2012) Cancer treatment and survivorship statistics. CA: A Can- cer Journal for Clinicians, 62, 220-241. http://dx.doi.org/10.3322/caac.21149 [3] Ferguson, N.L., Bell, J., Heidel, R., Lee, S., Vanmete, S, Duncan, L., Munsey, B., Panella, T. and Orucevic, A. (2013) Prognostic value of breast cancer subtypes, Ki-67 proliferation index, age, and pathologic tumor character- istics on breast cancer survival in Caucasian women. Breast Journal, 19, 22-30. http://dx.doi.org/10.1111/tbj.12059 [4] Buijs, J.T. and Pluijm, G. (2009) Osteotropic cancers: From primary tumor to bone. Cancer Letters, 273, 177- 193. http://dx.doi.org/10.1016/j.canlet.2008.05.044 [5] Coleman, R.E. (1997) Skeletal complications of malig- nancy. Cancer, 80, 1588-1594. [6] Mundy, G.R. (2002) Metastasis to bone: Causes, conse- quences and therapeutic opportunities. Nature Reviews Cancer, 2, 584-593. http://dx.doi.org/10.1038/nrc867 [7] Oster, G., Lamerato, L., Glass, A.G., Richert-Boe, K.E., Lopez, A., Chung, K., Richhariya, A., Dodge, T., Wolff, G.G., Balakumaran, A. and Edelsberg, J. (2013) Natural history of skeletal-related events in patients with breast, Copyright © 2013 SciRes. OPEN ACCESS
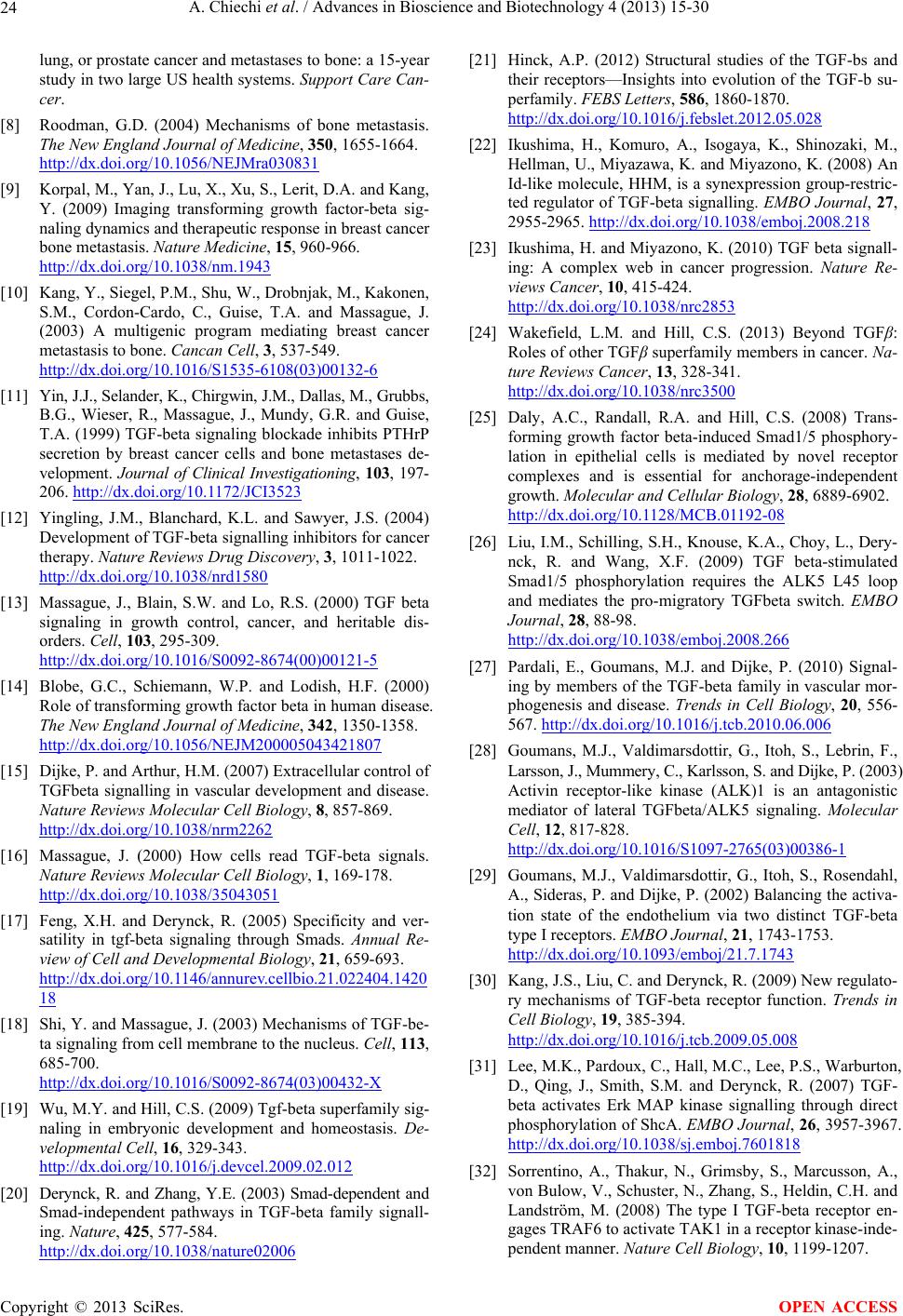 A. Chiechi et al. / Advances in Bioscience and Biotechnology 4 (2013) 15-30 24 lung, or prostate cancer and metastases to bone: a 15-year study in two large US health systems. Support Care Can- cer. [8] Roodman, G.D. (2004) Mechanisms of bone metastasis. The New England Journal of Medicine, 350, 1655-1664. http://dx.doi.org/10.1056/NEJMra030831 [9] Korpal, M., Yan, J., Lu, X., Xu, S., Lerit, D.A. and Kang, Y. (2009) Imaging transforming growth factor-beta sig- naling dynamics and therapeutic response in breast cancer bone metastasis. Nature Medicine, 15, 960-966. http://dx.doi.org/10.1038/nm.1943 [10] Kang, Y., Siegel, P.M., Shu, W., Drobnjak, M., Kakonen, S.M., Cordon-Cardo, C., Guise, T.A. and Massague, J. (2003) A multigenic program mediating breast cancer metastasis to bone. Cancan Cell, 3, 537-549. http://dx.doi.org/10.1016/S1535-6108(03)00132-6 [11] Yin, J.J., Selander, K., Chirgwin, J.M., Dallas, M., Grubbs, B.G., Wieser, R., Massague, J., Mundy, G.R. and Guise, T.A. (1999) TGF-beta signaling blockade inhibits PTHrP secretion by breast cancer cells and bone metastases de- velopment. Journal of Clinical Investigationing, 103, 197- 206. http://dx.doi.org/10.1172/JCI3523 [12] Yingling, J.M., Blanchard, K.L. and Sawyer, J.S. (2004) Development of TGF-beta signalling inhibitors for cancer therapy. Nature Reviews Drug Discovery, 3, 1011-1022. http://dx.doi.org/10.1038/nrd1580 [13] Massague, J., Blain, S.W. and Lo, R.S. (2000) TGF beta signaling in growth control, cancer, and heritable dis- orders. Cell, 103, 295-309. http://dx.doi.org/10.1016/S0092-8674(00)00121-5 [14] Blobe, G.C., Schiemann, W.P. and Lodish, H.F. (2000) Role of transforming growth factor beta in human disease. The New England Journal of Medicine, 342, 1350-1358. http://dx.doi.org/10.1056/NEJM200005043421807 [15] Dijke, P. and Arthur, H.M. (2007) Extracellular control of TGFbeta signalling in vascular development and disease. Nature Reviews Molecular Cell Biology, 8, 857-869. http://dx.doi.org/10.1038/nrm2262 [16] Massague, J. (2000) How cells read TGF-beta signals. Nature Reviews Molecular Cell Biology, 1, 169-178. http://dx.doi.org/10.1038/35043051 [17] Feng, X.H. and Derynck, R. (2005) Specificity and ver- satility in tgf-beta signaling through Smads. Annual Re- view of Cell and Developmental Biology, 21, 659-693. http://dx.doi.org/10.1146/annurev.cellbio.21.022404.1420 18 [18] Shi, Y. and Massague, J. (2003) Mechanisms of TGF-be- ta signaling from cell membrane to the nucleus. Cell, 113, 685-700. http://dx.doi.org/10.1016/S0092-8674(03)00432-X [19] Wu, M.Y. and Hill, C.S. (2009) Tgf-beta superfamily sig- naling in embryonic development and homeostasis. De- velopmental Cell, 16, 329-343. http://dx.doi.org/10.1016/j.devcel.2009.02.012 [20] Derynck, R. and Zhang, Y.E. (2003) Smad-dependent and Smad-independent pathways in TGF-beta family signall- ing. Nature, 425, 577-584. http://dx.doi.org/10.1038/nature02006 [21] Hinck, A.P. (2012) Structural studies of the TGF-bs and their receptors—Insights into evolution of the TGF-b su- perfamily. FEBS Letters, 586, 1860-1870. http://dx.doi.org/10.1016/j.febslet.2012.05.028 [22] Ikushima, H., Komuro, A., Isogaya, K., Shinozaki, M., Hellman, U., Miyazawa, K. and Miyazono, K. (2008) An Id-like molecule, HHM, is a synexpression group-restric- ted regulator of TGF-beta signalling. EMBO Journal, 27, 2955-2965. http://dx.doi.org/10.1038/emboj.2008.218 [23] Ikushima, H. and Miyazono, K. (2010) TGF beta signall- ing: A complex web in cancer progression. Nature Re- views Cancer, 10, 415-424. http://dx.doi.org/10.1038/nrc2853 [24] Wakefield, L.M. and Hill, C.S. (2013) Beyond TGFβ: Roles of other TGFβ superfamily members in cancer. Na- ture Reviews Cancer, 13, 328-341. http://dx.doi.org/10.1038/nrc3500 [25] Daly, A.C., Randall, R.A. and Hill, C.S. (2008) Trans- forming growth factor beta-induced Smad1/5 phosphory- lation in epithelial cells is mediated by novel receptor complexes and is essential for anchorage-independent growth. Molecular and Cellular Biology, 28, 6889-6902. http://dx.doi.org/10.1128/MCB.01192-08 [26] Liu, I.M., Schilling, S.H., Knouse, K.A., Choy, L., Dery- nck, R. and Wang, X.F. (2009) TGF beta-stimulated Smad1/5 phosphorylation requires the ALK5 L45 loop and mediates the pro-migratory TGFbeta switch. EMBO Journal, 28, 88-98. http://dx.doi.org/10.1038/emboj.2008.266 [27] Pardali, E., Goumans, M.J. and Dijke, P. (2010) Signal- ing by members of the TGF-beta family in vascular mor- phogenesis and disease. Trends in Cell Biology, 20, 556- 567. http://dx.doi.org/10.1016/j.tcb.2010.06.006 [28] Goumans, M.J., Valdimarsdottir, G., Itoh, S., Lebrin, F., Larsson, J., Mummery, C., Karlsson, S. and Dijke, P. (2003) Activin receptor-like kinase (ALK)1 is an antagonistic mediator of lateral TGFbeta/ALK5 signaling. Molecular Cell, 12, 817-828. http://dx.doi.org/10.1016/S1097-2765(03)00386-1 [29] Goumans, M.J., Valdimarsdottir, G., Itoh, S., Rosendahl, A., Sideras, P. and Dijke, P. (2002) Balancing the activa- tion state of the endothelium via two distinct TGF-beta type I receptors. EMBO Journal, 21, 1743-1753. http://dx.doi.org/10.1093/emboj/21.7.1743 [30] Kang, J.S., Liu, C. and Derynck, R. (2009) New regulato- ry mechanisms of TGF-beta receptor function. Trends in Cell Biology, 19, 385-394. http://dx.doi.org/10.1016/j.tcb.2009.05.008 [31] Lee, M.K., Pardoux, C., Hall, M.C., Lee, P.S., Warburton, D., Qing, J., Smith, S.M. and Derynck, R. (2007) TGF- beta activates Erk MAP kinase signalling through direct phosphorylation of ShcA. EMBO Journal, 26, 3957-3967. http://dx.doi.org/10.1038/sj.emboj.7601818 [32] Sorrentino, A., Thakur, N., Grimsby, S., Marcusson, A., von Bulow, V., Schuster, N., Zhang, S., Heldin, C.H. and Landström, M. (2008) The type I TGF-beta receptor en- gages TRAF6 to activate TAK1 in a receptor kinase-inde- pendent manner. Nature Cell Biology, 10, 1199-1207. Copyright © 2013 SciRes. OPEN ACCESS
 A. Chiechi et al. / Advances in Bioscience and Biotechnology 4 (2013) 15-30 25 http://dx.doi.org/10.1038/ncb1780 [33] Yamashita, M., Fatyol, K., Jin, C., Wang, X., Liu, Z. and Zhang, Y.E. (2008) TRAF6 mediates Smad-independent activation of JNK and p38 by TGF-beta. Molecular Cell, 31, 918-924. http://dx.doi.org/10.1016/j.molcel.2008.09.002 [34] Vergara, D., Merlot, B., Lucot, J.P., Collinet, P., Vinatier, D., Fournier, I. and Salzet, M. (2010) Epithelial-mesen- chymal transition in ovarian cancer. Cancer Letter, 291, 59-66. http://dx.doi.org/10.1016/j.canlet.2009.09.017 [35] Xu, J., Lamouille, S. and Derynck, R. (2009) TGF-β-in- duced epithelial to mesenchymal transition. Cell Re- search, 19, 156-172. http://dx.doi.org/10.1038/cr.2009.5 [36] Moustakas, A. and Heldin, C.H. (2007) Signaling net- works guiding epithelial-mesenchymal transitions during embryogenesis and cancer progression. Cancer Science, 98, 1512-1520. http://dx.http://dx.doi.org/10.1111/j.1349-7006.2007.005 50.x [37] Vincent, T., Neve, E.P., Johnson, J.R., Kukalev, A., Rojo, F., Albanell, J., Pietras, K., Virtanen, I., Philipson, L., Leopold, P.L., Crystal, R.G., de Herreros, A.G., Mousta- kas, A., Pettersson, R.F. and Fuxe, J. (2009) A SNAIL1- SMAD3/4 transcriptional repressor complex promotes TGF-b mediated epithelial-mesenchymal transition. Na- ture Cell Biology, 11, 943-950. http://dx.http://dx.doi.org/10.1038/ncb1905 [38] Bracken, C.P., Gregory, P.A., Kolesnikoff, N., Bert, A.G., Wang, J., Shannon, M.F. and Goodall, G.J. (2008) A double-negative feedback loop between ZEB1-SIP1 and the microRNA-200 family regulates epithelial-mesen- chymal transition. Cancer Research, 68, 7846-7854. http://dx.http://dx.doi.org/10.1158/0008-5472.CAN-08-1 942 [39] Moustakas, A. and Heldin, C.H. (2012) Induction of epi- thelial-mesenchymal transition by transforming growth factor beta. Seminars in Cancer Biology, 22, 446-454. http://dx.http://dx.doi.org/10.1016/j.semcance r.2012.04.002 [40] Horiguchi, K., Sakamoto, K., Koinuma, D., Semba, K., Inoue, A., Inoue, S., Fujii, H., Yamaguchi, A., Miyazawa, K., Miyazono, K. and Saitoh, M. (2012) TGF-β drives epithelial-mesenchymal transition through deltaEF1-me- diated downregulation of ESRP. Oncogene, 31 , 3190- 3201. http://dx.http://dx.doi.org/10.1038/onc.2011.493 [41] Ozdamar, B., Bose, R., Barrios-Rodiles, M., Wang, H.R., Zhang, Y. and Wrana, J.L. (2005) Regulation of the po- larity protein Par6 by TGFβ receptors controls epithelial cell plasticity. Science, 307, 1603-1609. http://dx.http://dx.doi.org/10.1126/science.1105718 [42] Katsuno, Y., Lamouille, S. and Derynck, R. (2013) TGF-β signaling and epithelial-mesenchymal transition in cancer progression. Current Opinion in Oncology, 25, 76-84. http://dx.http://dx.doi.org/10.1097/CCO.0b013e32835b6371 [43] Netherton, S.J. and Bonni, S. (2010) Suppression of TGFβ-induced epithelial-mesenchymal transition like phenotype by a PIAS1 regulated sumoylation pathway in NMuMG epithelial cells. PLoS ONE, 5, e13971. http://dx.http://dx.doi.org/10.1371/journal.pone.0013971 [44] Derynck, R. and Akhurst, R.J. (2007) Differentiation plasticity regulated by TGF-beta family proteins in deve- lopment and disease. Nature Cell Biology, 9, 1000-1004. http://dx.http://dx.doi.org/10.1038/ncb434 [45] Massague, J. and Chen, Y.G. (2000) Controlling TGF- beta signaling. Genes & Development, 14, 627-644. [46] Massague, J. (2008) TGFbeta in Cancer. Cell, 134, 215- 230. http://dx.http://dx.doi.org/10.1016/j.cell.2008.07.001 [47] Thiery, J.P., Acloque, H., Huang, R.Y. and Nieto, M.A. (2009) Epithelial-mesenchymal transitions in develop- ment and disease. Cell, 139, 871-890. http://dx.http://dx.doi.org/10.1016/j.cell.2009.11.007 [48] Yang, Y.A., Dukhanina, O., Tang, B., Mamura, M., Letterio, J.J., MacGregor, J., Patel, S.C., Khozin, S., Liu, Z.Y., Green, J., Anver, M.R., Merlino, G. and Wakefield, L.M. (2002) Lifetime exposure to a soluble TGF-beta an- tagonist protects mice against metastasis without adverse side effects. Journal of Clinical Investigation, 109, 1607-1615. [49] Gorska, A.E., Jensen, R.A., Shyr, Y., Aakre, M.E., Bhowmick, N.A. and Moses, H.L. (2003) Transgenic mice expressing a dominant-negative mutant type II trans- forming growth factor-beta receptor exhibit impaired mammary development and enhanced mammary tumor formation. American Journal of Pathology, 63, 1539- 1549. http://dx.http://dx.doi.org/10.1016/S0002-9440(10)63510-9 [50] Lenferink, A.E., Magoon, J., Pepin, M.C., Guimond, A. and O’Connor-McCourt, M.D. (2003) Expression of TGF-beta type II receptor antisense RNA impairs TGF- beta signaling in vitro and promotes mammary gland differentiation in vivo. International Journal of Cancer, 107, 919-928. http://dx.http://dx.doi.org/10.1002/ijc.11494 [51] Muraoka, R.S., Koh, Y., Roebuck, L.R., Sanders, M.E., Brantley-Sieders, D., Gorska, A.E., Moses, H.L. and Ar- teaga, C.L. (2003) Increased malignancy of Neu-induced mammary tumors overexpressing active transforming growth factor beta1. Molecular and Cellular Biology, 23, 8691-8703. http://dx.http://dx.doi.org/10.1128/MCB.23.23.8691-8703 .2003 [52] Siegel, P.M., Shu, W., Cardiff, R.D., Muller, W.J. and Massague, J. (2003) Transforming growth factor beta sig- naling impairs Neu-induced mammary tumorigenesis while promoting pulmonary metastasis. Proceedings of the National Academy of Sciences of the United States of America, 100, 8430-8435. http://dx.http://dx.doi.org/10.1073/pnas.0932636100 [53] Zakharchenko, O., Cojoc, M., Dubrovska, A. and Souchelnytskyi, S. (2013) A role of TGFβ1 dependent 14-3-3σ phosphorylation at Ser69 and Ser74 in the re- gulation of gene transcription, stemness and radio- resistance. PLoS ONE, 8, e65163. http://dx.http://dx.doi.org/10.1371/journal.pone.0065163 [54] Wang, Y. and Lui, W.Y. (2012) Transforming growth factor-β1 attenuates junctional adhesion molecule-A and contributes to breast cancer cell invasion. European Jour- nal of Cancer, 48, 3475-3487. http://dx.http://dx.doi.org/10.1016/j.ejca.2012.04.016 Copyright © 2013 SciRes. OPEN ACCESS
 A. Chiechi et al. / Advances in Bioscience and Biotechnology 4 (2013) 15-30 26 [55] Johansson, J., Berg, T., Kurzejamska, E., Pan, M.F., Tabor, V., Jansson, M., Roswall, P., Pietras, K., Sund, M., Religa, P. and Fuxe. J. (2013) MiR-155-mediated loss of C/EBPβ shifts the TGF-β response from growth inhi- bition to epithelial-mesenchymal transition, invasion and metastasis in breast cancer. Oncogene. [56] Forrester, E., Chytil, A., Bierie, B., Aakre, M., Gorska, A.E., Sharif-Afshar, A.R., Muller, W.J. and Moses, H.L. (2005) Effect of conditional knockout of the type II TGF- beta receptor gene in mammary epithelia on mammary gland development and polyomavirus middle T antigen induced tumor formation and metastasis. Cancer Resear- ch, 65, 2296-2302. http://dx.http://dx.doi.org/10.1158/0008-5472.CAN-04-3 272 [57] Muraoka-Cook, R.S., Shin, I., Yi, J.Y., Easterly, E., Barcellos-Hoff, M.H., Yingling, J.M., Zent, R. and Ar- teaga, C.L. (2006) Activated type I TGFbeta receptor kinase enhances the survival of mammary epithelial cells and accelerates tumor progression. Oncogene, 25, 3408- 3423. http://dx.http://dx.doi.org/10.1038/sj.onc.1208964 [58] Gorsch, S.M., Memoli, V.A., Stukel, T.A., Gold, L.I. and Arrick, B.A. (1992) Immunohistochemical staining for transforming growth factor beta 1 associates with disease progression in human breast cancer. Cancer Research, 52, 6949-6952. [59] Tan, A.R., Alexe, G. and Reiss, M. (2009) Transforming growth factor-beta signaling: emerging stem cell target in metastatic breast cancer? Breast Cancer Research and Treatment, 115, 453-495. http://dx.http://dx.doi.org/10.1007/s10549-008-0184-1 [60] Travers, M.T., Barrett-Lee, P.J., Berger, U., Luqmani, Y.A., Gazet, J.C., Powles, T.J. and Coombes RC. (1988) Growth factor expression in normal, benign, and malig- nant breast tissue. British Medical Journal, 296, 1621- 1624. http://dx.http://dx.doi.org/10.1136/bmj.296.6637.1621 [61] Jong, J.S., Diest, P.J., Valk, P. and Baak, J.P. (1998) Expression of growth factors, growth-inhibiting factors, and their receptors in invasive breast cancer. II: Cor- relations with proliferation and angiogenesis. The Journal of Pathology, 184, 53-57. http://dx.http://dx.doi.org/10.1002/(SICI)1096-9896(1998 01)184:1<53::AID-PATH6>3.0.CO;2-7 [62] Grau, A.M., Wen, W., Ramroopsingh, D.S., Gao, Y.T., Zi, J., Cai, Q., Shu, X.O. and Zheng, W. (2008) Circulating transforming growth factor-beta-1 and breast cancer prognosis: results from the Shanghai Breast Cancer Study. Breast Cancer Research and Treatment, 112, 335-341. http://dx.http://dx.doi.org/10.1007/s10549-007-9845-8 [63] Ivanovic, V., Todorovic-Rakovic, N., Demajo, M., Neskovic-Konstantinovic, Z., Subota, V., Ivanisevic-Mi- lovanovic, O. and Nikolic-Vukosavljevic, D. (2003) Ele- vated plasma levels of transforming growth factor-beta 1 (TGF-beta 1) in patients with advanced breast cancer: association with disease progression. European Journal of Cancer, 39, 454-461. http://dx.http://dx.doi.org/10.1016/S0959-8049(02)00502-6 [64] Kong, F.M., Anscher, M.S., Murase, T., Abbott, B.D., Iglehart, J.D. and Jirtle, R.L. (1995) Elevated plasma transforming growth factor-beta 1 levels in breast cancer patients decrease after surgical removal of the tumor. An- nals of Surgery, 222, 155-162. http://dx.http://dx.doi.org/10.1097/00000658-199508000- 00007 [65] Sheen-Chen, S.M., Chen, H.S., Sheen, C.W., Eng, H.L. and Chen, W.J. (2001) Serum levels of transforming growth factor beta1 in patients with breast cancer. Archi- ves of Surgery, 136, 937-940. http://dx.http://dx.doi.org/10.1001/archsurg.136.8.937 [66] Baselga, J., Rothenberg, M.L., Tabernero, J., Seoane, J., Daly, T., Cleverly, A., Berry, B., Rhoades, S.K., Ray, C.A., Fill, J., Farrington, D.L., Wallace, L. A., Yingling, J.M., Lahn, M., Arteaga, C. and Carducci, M. (2008) TGF-beta signalling-related markers in cancer patients with bone metastasis. Biomarkers, 13, 217-236. http://dx.http://dx.doi.org/10.1080/13547500701676019 [67] Divella, R., Daniele, A., Savino, E., Palma, F., Bellizzi, A., Giotta, F., Simone, G., Lioce, M., Quaranta, M., Paradiso, A. and Mazzocca, A. (2013) Circulating levels of transforming growth factor-βeta (TGF-β) and chemo- kine (C-X-C motif) ligand-1 (CXCL1) as predictors of distant seeding of circulating tumor cells in patients with metastatic breast cancer. Anticancer Research, 33, 1491- 1497. [68] Buck, M.B., Fritz, P., Dippon, J., Zugmaie, R.G. and Knabbe, C. (2004) Prognostic significance of transform- ing growth factor beta receptor II in estrogen receptor- negative breast cancer patients. Clinical Cancer Research, 10, 491-498. http://dx.http://dx.doi.org/10.1158/1078-0432. CCR-0320-03 [69] Richardsen, E., Uglehus, R.D., Johnsen, S.H. and Busund, L.T. (2012) Immunohistochemical expression of epithet- lial and stromal immunomodulatory signalling molecules is a prognostic indicator in breast cancer. BMC Research Notes, 21, 110. http://dx.http://dx.doi.org/10.1186/1756-0500-5-110 [70] de Kruijf, E.M., Dekker, T.J., Hawinkels, L.J., Putter, H., Smit, V.T., Kroep, J.R., Kuppen, P.J., van de Velde, C.J., ten Dijke, P., Tollenaar, R.A. and Mesker, W.E. (2013) The prognostic role of TGF-β signaling pathway in breast cancer patients. Ann Oncol, 24, 384-390. http://dx.http://dx.doi.org/10.1093/annonc/mds333 [71] Al-Hajj, M., Wicha, M.S., Benito-Hernandez, A., Morri- son, S.J. and Clarke, M.F. (2003) Prospective identifica- tion of tumorigenic breast cancer cells. Proceedings of the National Academy of Sciences of the United States of America, 100, 3983-3988. http://dx.http://dx.doi.org/10.1073/pnas.0530291100 [72] Collins, A.T., Berry, P.A., Hyde, C., Stower, M.J. and Maitland, N.J. (2005) Prospective identification of tumo- rigenic prostate cancer stem cells. Cancer Research, 65, 10946-10951. http://dx.http://dx.doi.org/10.1158/0008-5472.CAN-05-2 018 [73] O’Brien, C.A., Pollett, A., Gallinger, S. and Dick, J.E. (2007) A human colon cancer cell capable of initiating tumour growth in immunodeficient mice. Nature, 445, 106-110. http://dx.http://dx.doi.org/10.1038/nature05372 [74] Reya, T., Morrison, S.J., Clarke, M.F. and Weissman, I.L. Copyright © 2013 SciRes. OPEN ACCESS
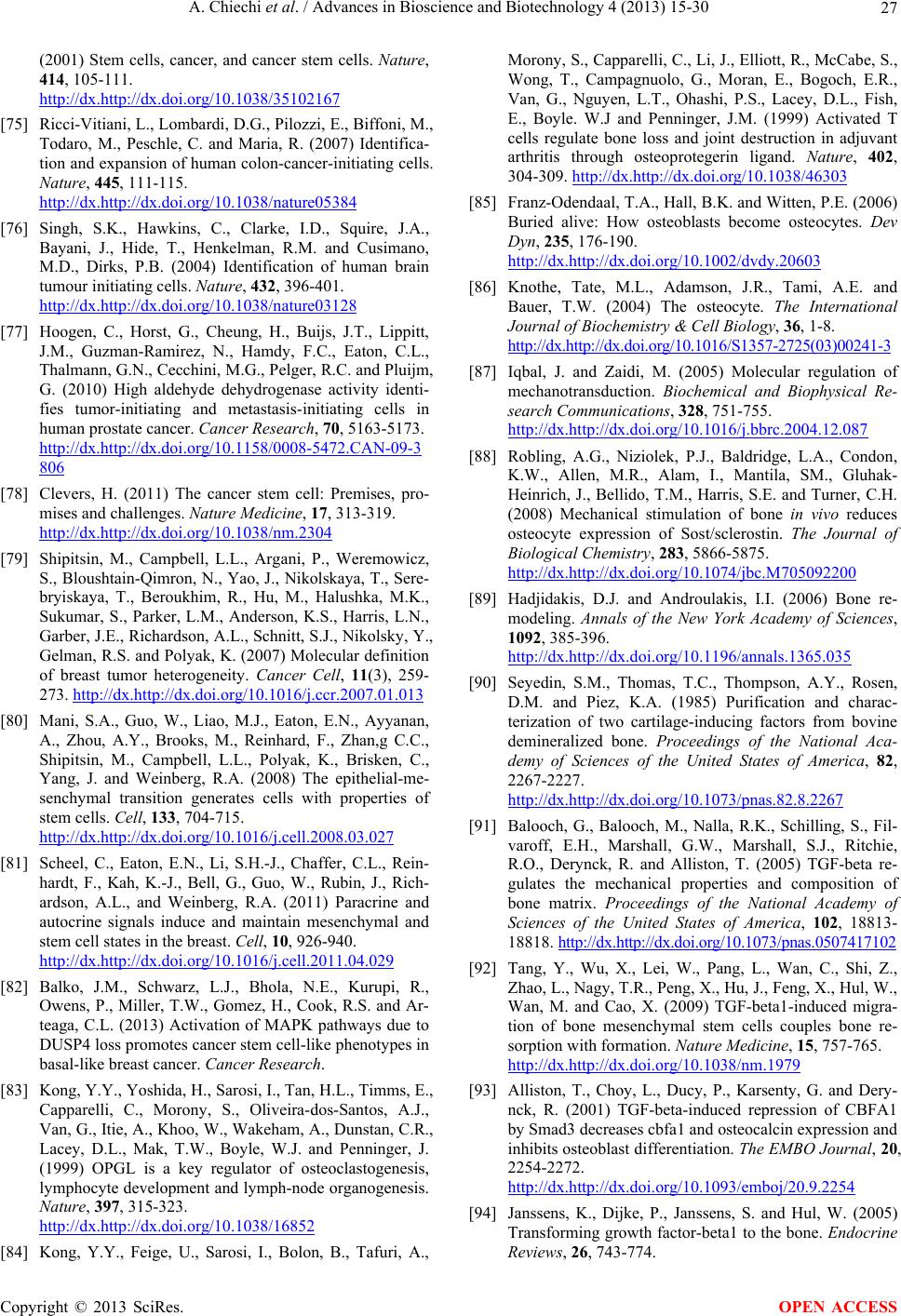 A. Chiechi et al. / Advances in Bioscience and Biotechnology 4 (2013) 15-30 27 (2001) Stem cells, cancer, and cancer stem cells. Nature, 414, 105-111. http://dx.http://dx.doi.org/10.1038/35102167 [75] Ricci-Vitiani, L., Lombardi, D.G., Pilozzi, E., Biffoni, M., Todaro, M., Peschle, C. and Maria, R. (2007) Identifica- tion and expansion of human colon-cancer-initiating cells. Nature, 445, 111-115. http://dx.http://dx.doi.org/10.1038/nature05384 [76] Singh, S.K., Hawkins, C., Clarke, I.D., Squire, J.A., Bayani, J., Hide, T., Henkelman, R.M. and Cusimano, M.D., Dirks, P.B. (2004) Identification of human brain tumour initiating cells. Nature, 432, 396-401. http://dx.http://dx.doi.org/10.1038/nature03128 [77] Hoogen, C., Horst, G., Cheung, H., Buijs, J.T., Lippitt, J.M., Guzman-Ramirez, N., Hamdy, F.C., Eaton, C.L., Thalmann, G.N., Cecchini, M.G., Pelger, R.C. and Pluijm, G. (2010) High aldehyde dehydrogenase activity identi- fies tumor-initiating and metastasis-initiating cells in human prostate cancer. Cancer Research, 70, 5163-5173. http://dx.http://dx.doi.org/10.1158/0008-5472.CAN-09-3 806 [78] Clevers, H. (2011) The cancer stem cell: Premises, pro- mises and challenges. Nature Medicine, 17, 313-319. http://dx.http://dx.doi.org/10.1038/nm.2304 [79] Shipitsin, M., Campbell, L.L., Argani, P., Weremowicz, S., Bloushtain-Qimron, N., Yao, J., Nikolskaya, T., Sere- bryiskaya, T., Beroukhim, R., Hu, M., Halushka, M.K., Sukumar, S., Parker, L.M., Anderson, K.S., Harris, L.N., Garber, J.E., Richardson, A.L., Schnitt, S.J., Nikolsky, Y., Gelman, R.S. and Polyak, K. (2007) Molecular definition of breast tumor heterogeneity. Cancer Cell, 11(3), 259- 273. http://dx.http://dx.doi.org/10.1016/j.ccr.2007.01.013 [80] Mani, S.A., Guo, W., Liao, M.J., Eaton, E.N., Ayyanan, A., Zhou, A.Y., Brooks, M., Reinhard, F., Zhan,g C.C., Shipitsin, M., Campbell, L.L., Polyak, K., Brisken, C., Yang, J. and Weinberg, R.A. (2008) The epithelial-me- senchymal transition generates cells with properties of stem cells. Cell, 133, 704-715. http://dx.http://dx.doi.org/10.1016/j.cell.2008.03.027 [81] Scheel, C., Eaton, E.N., Li, S.H.-J., Chaffer, C.L., Rein- hardt, F., Kah, K.-J., Bell, G., Guo, W., Rubin, J., Rich- ardson, A.L., and Weinberg, R.A. (2011) Paracrine and autocrine signals induce and maintain mesenchymal and stem cell states in the breast. Cell, 10, 926-940. http://dx.http://dx.doi.org/10.1016/j.cell.2011.04.029 [82] Balko, J.M., Schwarz, L.J., Bhola, N.E., Kurupi, R., Owens, P., Miller, T.W., Gomez, H., Cook, R.S. and Ar- teaga, C.L. (2013) Activation of MAPK pathways due to DUSP4 loss promotes cancer stem cell-like phenotypes in basal-like breast cancer. Cancer Research. [83] Kong, Y.Y., Yoshida, H., Sarosi, I., Tan, H.L., Timms, E., Capparelli, C., Morony, S., Oliveira-dos-Santos, A.J., Van, G., Itie, A., Khoo, W., Wakeham, A., Dunstan, C.R., Lacey, D.L., Mak, T.W., Boyle, W.J. and Penninger, J. (1999) OPGL is a key regulator of osteoclastogenesis, lymphocyte development and lymph-node organogenesis. Nature, 397, 315-323. http://dx.http://dx.doi.org/10.1038/16852 [84] Kong, Y.Y., Feige, U., Sarosi, I., Bolon, B., Tafuri, A., Morony, S., Capparelli, C., Li, J., Elliott, R., McCabe, S., Wong, T., Campagnuolo, G., Moran, E., Bogoch, E.R., Van, G., Nguyen, L.T., Ohashi, P.S., Lacey, D.L., Fish, E., Boyle. W.J and Penninger, J.M. (1999) Activated T cells regulate bone loss and joint destruction in adjuvant arthritis through osteoprotegerin ligand. Nature, 402, 304-309. http://dx.http://dx.doi.org/10.1038/46303 [85] Franz-Odendaal, T.A., Hall, B.K. and Witten, P.E. (2006) Buried alive: How osteoblasts become osteocytes. Dev Dyn, 235, 176-190. http://dx.http://dx.doi.org/10.1002/dvdy.20603 [86] Knothe, Tate, M.L., Adamson, J.R., Tami, A.E. and Bauer, T.W. (2004) The osteocyte. The International Journal of Biochemistry & Cell Biology, 36, 1-8. http://dx.http://dx.doi.org/10.1016/S1357-2725(03)00241-3 [87] Iqbal, J. and Zaidi, M. (2005) Molecular regulation of mechanotransduction. Biochemical and Biophysical Re- search Communications, 328, 751-755. http://dx.http://dx.doi.org/10.1016/j.bbrc.2004.12.087 [88] Robling, A.G., Niziolek, P.J., Baldridge, L.A., Condon, K.W., Allen, M.R., Alam, I., Mantila, SM., Gluhak- Heinrich, J., Bellido, T.M., Harris, S.E. and Turner, C.H. (2008) Mechanical stimulation of bone in vivo reduces osteocyte expression of Sost/sclerostin. The Journal of Biological Chemistry, 283, 5866-5875. http://dx.http://dx.doi.org/10.1074/jbc.M705092200 [89] Hadjidakis, D.J. and Androulakis, I.I. (2006) Bone re- modeling. Annals of the New York Academy of Sciences, 1092, 385-396. http://dx.http://dx.doi.org/10.1196/annals.1365.035 [90] Seyedin, S.M., Thomas, T.C., Thompson, A.Y., Rosen, D.M. and Piez, K.A. (1985) Purification and charac- terization of two cartilage-inducing factors from bovine demineralized bone. Proceedings of the National Aca- demy of Sciences of the United States of America, 82, 2267-2227. http://dx.http://dx.doi.org/10.1073/pnas.82.8.2267 [91] Balooch, G., Balooch, M., Nalla, R.K., Schilling, S., Fil- varoff, E.H., Marshall, G.W., Marshall, S.J., Ritchie, R.O., Derynck, R. and Alliston, T. (2005) TGF-beta re- gulates the mechanical properties and composition of bone matrix. Proceedings of the National Academy of Sciences of the United States of America, 102, 18813- 18818. http://dx.http://dx.doi.org/10.1073/pna s.0507417102 [92] Tang, Y., Wu, X., Lei, W., Pang, L., Wan, C., Shi, Z., Zhao, L., Nagy, T.R., Peng, X., Hu, J., Feng, X., Hul, W., Wan, M. and Cao, X. (2009) TGF-beta1-induced migra- tion of bone mesenchymal stem cells couples bone re- sorption with formation. Nature Medicine, 15, 757-765. http://dx.http://dx.doi.org/10.1038/nm.1979 [93] Alliston, T., Choy, L., Ducy, P., Karsenty, G. and Dery- nck, R. (2001) TGF-beta-induced repression of CBFA1 by Smad3 decreases cbfa1 and osteocalcin expression and inhibits osteoblast differentiation. The EMBO Journal, 20, 2254-2272. http://dx.http://dx.doi.org/10.1093/emboj/20.9.2254 [94] Janssens, K., Dijke, P., Janssens, S. and Hul, W. (2005) Transforming growth factor-beta1 to the bone. Endocrine Reviews, 26, 743-774. Copyright © 2013 SciRes. OPEN ACCESS
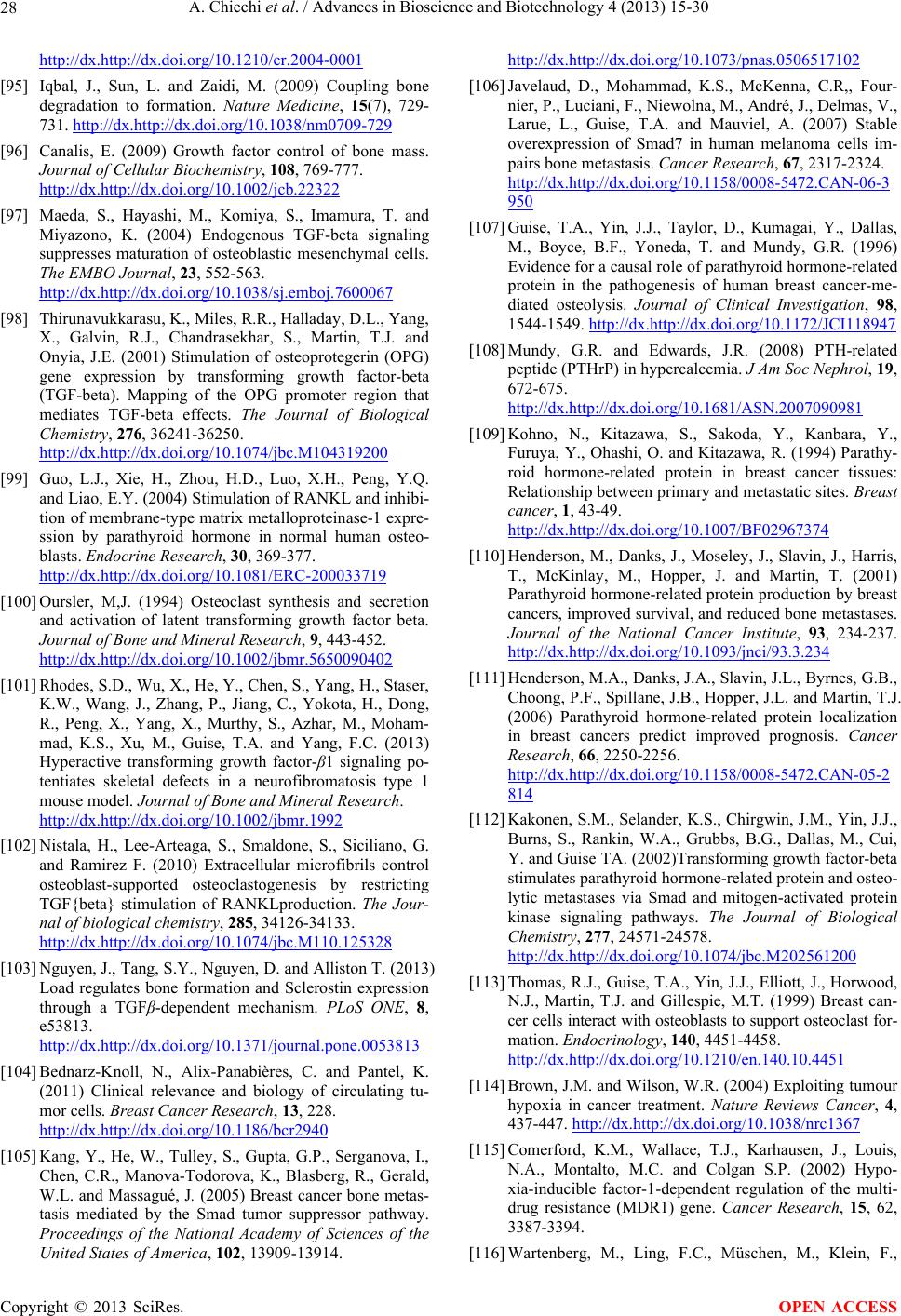 A. Chiechi et al. / Advances in Bioscience and Biotechnology 4 (2013) 15-30 28 http://dx.http://dx.doi.org/10.1210/er.2004-0001 [95] Iqbal, J., Sun, L. and Zaidi, M. (2009) Coupling bone degradation to formation. Nature Medicine, 15(7), 729- 731. http://dx.http://dx.doi.org/10.1038/nm0709-729 [96] Canalis, E. (2009) Growth factor control of bone mass. Journal of Cellular Biochemistry, 108, 769-777. http://dx.http://dx.doi.org/10.1002/jcb.22322 [97] Maeda, S., Hayashi, M., Komiya, S., Imamura, T. and Miyazono, K. (2004) Endogenous TGF-beta signaling suppresses maturation of osteoblastic mesenchymal cells. The EMBO Journal, 23, 552-563. http://dx.http://dx.doi.org/10.1038/sj.emboj.7600067 [98] Thirunavukkarasu, K., Miles, R.R., Halladay, D.L., Yang, X., Galvin, R.J., Chandrasekhar, S., Martin, T.J. and Onyia, J.E. (2001) Stimulation of osteoprotegerin (OPG) gene expression by transforming growth factor-beta (TGF-beta). Mapping of the OPG promoter region that mediates TGF-beta effects. The Journal of Biological Chemistry, 276, 36241-36250. http://dx.http://dx.doi.org/10.1074/jbc.M104319200 [99] Guo, L.J., Xie, H., Zhou, H.D., Luo, X.H., Peng, Y.Q. and Liao, E.Y. (2004) Stimulation of RANKL and inhibi- tion of membrane-type matrix metalloproteinase-1 expre- ssion by parathyroid hormone in normal human osteo- blasts. Endocrine Research, 30, 369-377. http://dx.http://dx.doi.org/10.1081/ERC-200033719 [100] Oursler, M,J. (1994) Osteoclast synthesis and secretion and activation of latent transforming growth factor beta. Journal of Bone and Mineral Research, 9, 443-452. http://dx.http://dx.doi.org/10.1002/jbmr.5650090402 [101] Rhodes, S.D., Wu, X., He, Y., Chen, S., Yang, H., Staser, K.W., Wang, J., Zhang, P., Jiang, C., Yokota, H., Dong, R., Peng, X., Yang, X., Murthy, S., Azhar, M., Moham- mad, K.S., Xu, M., Guise, T.A. and Yang, F.C. (2013) Hyperactive transforming growth factor-β1 signaling po- tentiates skeletal defects in a neurofibromatosis type 1 mouse model. Journal of Bone and Mineral Research. http://dx.http://dx.doi.org/10.1002/jbmr.1992 [102] Nistala, H., Lee-Arteaga, S., Smaldone, S., Siciliano, G. and Ramirez F. (2010) Extracellular microfibrils control osteoblast-supported osteoclastogenesis by restricting TGF{beta} stimulation of RANKLproduction. The Jour- nal of biological chemistry, 285, 34126-34133. http://dx.http://dx.doi.org/10.1074/jbc.M110.125328 [103] Nguyen, J., Tang, S.Y., Nguyen, D. and Alliston T. (2013) Load regulates bone formation and Sclerostin expression through a TGFβ-dependent mechanism. PLoS ONE, 8, e53813. http://dx.http://dx.doi.org/10.1371/journal.pone.0053813 [104] Bednarz-Knoll, N., Alix-Panabières, C. and Pantel, K. (2011) Clinical relevance and biology of circulating tu- mor cells. Breast Cancer Research, 13, 228. http://dx.http://dx.doi.org/10.1186/bcr2940 [105] Kang, Y., He, W., Tulley, S., Gupta, G.P., Serganova, I., Chen, C.R., Manova-Todorova, K., Blasberg, R., Gerald, W.L. and Massagué, J. (2005) Breast cancer bone metas- tasis mediated by the Smad tumor suppressor pathway. Proceedings of the National Academy of Sciences of the United States of America, 102, 13909-13914. http://dx.http://dx.doi.org/10.1073/pnas.0506517102 [106] Javelaud, D., Mohammad, K.S., McKenna, C.R,, Four- nier, P., Luciani, F., Niewolna, M., André, J., Delmas, V., Larue, L., Guise, T.A. and Mauviel, A. (2007) Stable overexpression of Smad7 in human melanoma cells im- pairs bone metastasis. Cancer Research, 67, 2317-2324. http://dx.http://dx.doi.org/10.1158/0008-5472.CAN-06-3 950 [107] Guise, T.A., Yin, J.J., Taylor, D., Kumagai, Y., Dallas, M., Boyce, B.F., Yoneda, T. and Mundy, G.R. (1996) Evidence for a causal role of parathyroid hormone-related protein in the pathogenesis of human breast cancer-me- diated osteolysis. Journal of Clinical Investigation, 98, 1544-1549. http://dx.http://dx.doi.org/10.1172/JCI118947 [108] Mundy, G.R. and Edwards, J.R. (2008) PTH-related peptide (PTHrP) in hypercalcemia. J Am Soc Nephrol, 19, 672-675. http://dx.http://dx.doi.org/10.1681/ASN.2007090981 [109] Kohno, N., Kitazawa, S., Sakoda, Y., Kanbara, Y., Furuya, Y., Ohashi, O. and Kitazawa, R. (1994) Parathy- roid hormone-related protein in breast cancer tissues: Relationship between primary and metastatic sites. Breast cancer, 1, 43-49. http://dx.http://dx.doi.org/10.1007/BF02967374 [110] Henderson, M., Danks, J., Moseley, J., Slavin, J., Harris, T., McKinlay, M., Hopper, J. and Martin, T. (2001) Parathyroid hormone-related protein production by breast cancers, improved survival, and reduced bone metastases. Journal of the National Cancer Institute, 93, 234-237. http://dx.http://dx.doi.org/10.1093/jnci/93.3.234 [111] Henderson, M.A., Danks, J.A., Slavin, J.L., Byrnes, G.B., Choong, P.F., Spillane, J.B., Hopper, J.L. and Martin, T.J. (2006) Parathyroid hormone-related protein localization in breast cancers predict improved prognosis. Cancer Research, 66, 2250-2256. http://dx.http://dx.doi.org/10.1158/0008-5472.CAN-05-2 814 [112] Kakonen, S.M., Selander, K.S., Chirgwin, J.M., Yin, J.J., Burns, S., Rankin, W.A., Grubbs, B.G., Dallas, M., Cui, Y. and Guise TA. (2002)Transforming growth factor-beta stimulates parathyroid hormone-related protein and osteo- lytic metastases via Smad and mitogen-activated protein kinase signaling pathways. The Journal of Biological Chemistry, 277, 24571-24578. http://dx.http://dx.doi.org/10.1074/jbc.M202561200 [113] Thomas, R.J., Guise, T.A., Yin, J.J., Elliott, J., Horwood, N.J., Martin, T.J. and Gillespie, M.T. (1999) Breast can- cer cells interact with osteoblasts to support osteoclast for- mation. Endocrinology, 140, 4451-4458. http://dx.http://dx.doi.org/10.1210/en.140.10.4451 [114] Brown, J.M. and Wilson, W.R. (2004) Exploiting tumour hypoxia in cancer treatment. Nature Reviews Cancer, 4, 437-447. http://dx.http://dx.doi.org/10.1038/nrc1367 [115] Comerford, K.M., Wallace, T.J., Karhausen, J., Louis, N.A., Montalto, M.C. and Colgan S.P. (2002) Hypo- xia-inducible factor-1-dependent regulation of the multi- drug resistance (MDR1) gene. Cancer Research, 15, 62, 3387-3394. [116] Wartenberg, M., Ling, F.C., Müschen, M., Klein, F., Copyright © 2013 SciRes. OPEN ACCESS
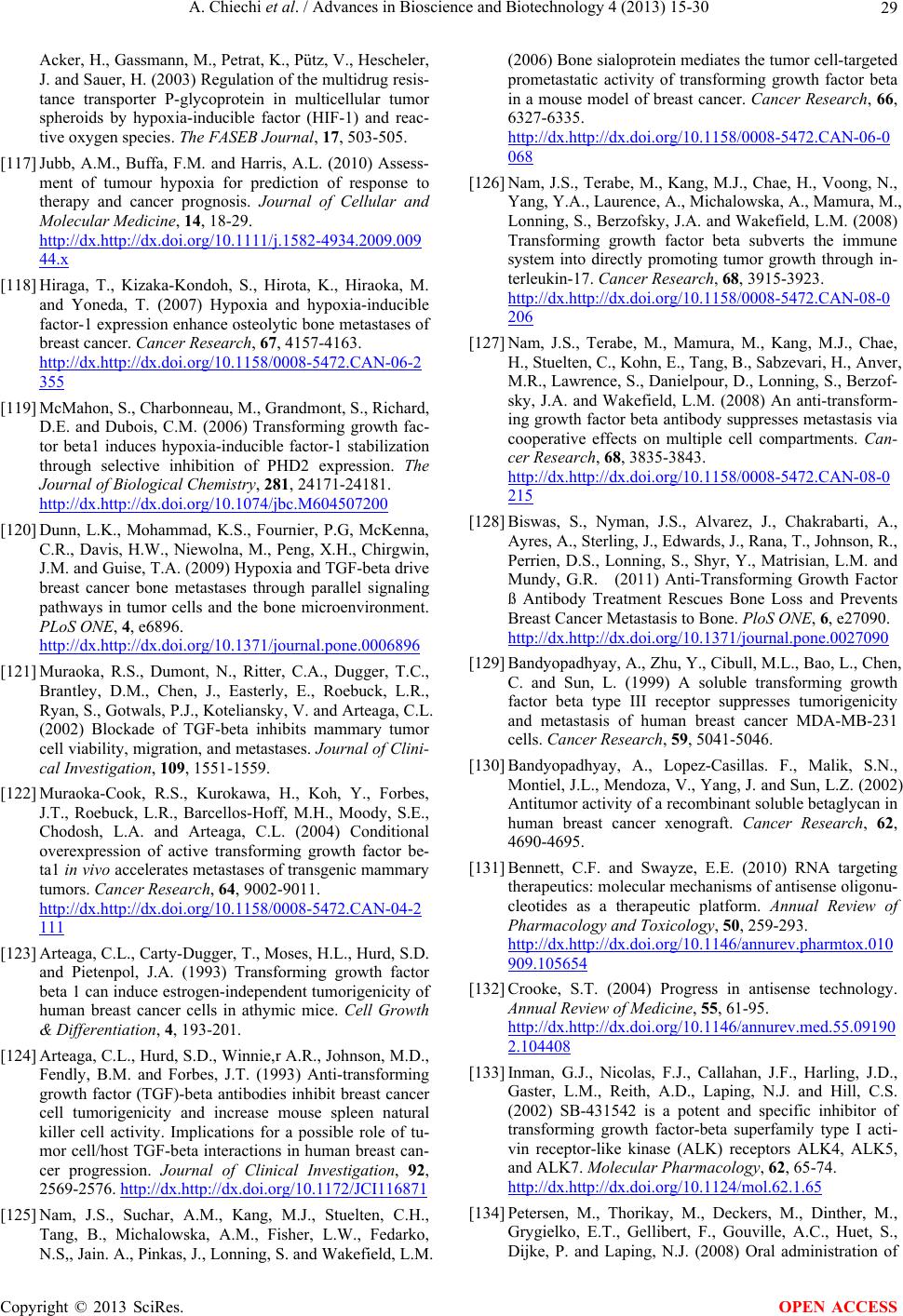 A. Chiechi et al. / Advances in Bioscience and Biotechnology 4 (2013) 15-30 29 Acker, H., Gassmann, M., Petrat, K., Pütz, V., Hescheler, J. and Sauer, H. (2003) Regulation of the multidrug resis- tance transporter P-glycoprotein in multicellular tumor spheroids by hypoxia-inducible factor (HIF-1) and reac- tive oxygen species. The FASEB Journal, 17, 503-505. [117] Jubb, A.M., Buffa, F.M. and Harris, A.L. (2010) Assess- ment of tumour hypoxia for prediction of response to therapy and cancer prognosis. Journal of Cellular and Molecular Medicine, 14, 18-29. http://dx.http://dx.doi.org/10.1111/j.1582-4934.2009.009 44.x [118] Hiraga, T., Kizaka-Kondoh, S., Hirota, K., Hiraoka, M. and Yoneda, T. (2007) Hypoxia and hypoxia-inducible factor-1 expression enhance osteolytic bone metastases of breast cancer. Cancer Research, 67, 4157-4163. http://dx.http://dx.doi.org/10.1158/0008-5472.CAN-06-2 355 [119] McMahon, S., Charbonneau, M., Grandmont, S., Richard, D.E. and Dubois, C.M. (2006) Transforming growth fac- tor beta1 induces hypoxia-inducible factor-1 stabilization through selective inhibition of PHD2 expression. The Journal of Biological Chemistry, 281, 24171-24181. http://dx.http://dx.doi.org/10.1074/jbc.M604507200 [120] Dunn, L.K., Mohammad, K.S., Fournier, P.G, McKenna, C.R., Davis, H.W., Niewolna, M., Peng, X.H., Chirgwin, J.M. and Guise, T.A. (2009) Hypoxia and TGF-beta drive breast cancer bone metastases through parallel signaling pathways in tumor cells and the bone microenvironment. PLoS ONE, 4, e6896. http://dx.http://dx.doi.org/10.1371/journal.pone.0006896 [121] Muraoka, R.S., Dumont, N., Ritter, C.A., Dugger, T.C., Brantley, D.M., Chen, J., Easterly, E., Roebuck, L.R., Ryan, S., Gotwals, P.J., Koteliansky, V. and Arteaga, C.L. (2002) Blockade of TGF-beta inhibits mammary tumor cell viability, migration, and metastases. Journal of Clini- cal Investigation, 109, 1551-1559. [122] Muraoka-Cook, R.S., Kurokawa, H., Koh, Y., Forbes, J.T., Roebuck, L.R., Barcellos-Hoff, M.H., Moody, S.E., Chodosh, L.A. and Arteaga, C.L. (2004) Conditional overexpression of active transforming growth factor be- ta1 in vivo accelerates metastases of transgenic mammary tumors. Cancer Research, 64, 9002-9011. http://dx.http://dx.doi.org/10.1158/0008-5472.CAN-04-2 111 [123] Arteaga, C.L., Carty-Dugger, T., Moses, H.L., Hurd, S.D. and Pietenpol, J.A. (1993) Transforming growth factor beta 1 can induce estrogen-independent tumorigenicity of human breast cancer cells in athymic mice. Cell Growth & Differentiation, 4, 193-201. [124] Arteaga, C.L., Hurd, S.D., Winnie,r A.R., Johnson, M.D., Fendly, B.M. and Forbes, J.T. (1993) Anti-transforming growth factor (TGF)-beta antibodies inhibit breast cancer cell tumorigenicity and increase mouse spleen natural killer cell activity. Implications for a possible role of tu- mor cell/host TGF-beta interactions in human breast can- cer progression. Journal of Clinical Investigation, 92, 2569-2576. http://dx.http://dx.doi.org/10.1172/JCI116871 [125] Nam, J.S., Suchar, A.M., Kang, M.J., Stuelten, C.H., Tang, B., Michalowska, A.M., Fisher, L.W., Fedarko, N.S,, Jain. A., Pinkas, J., Lonning, S. and Wakefield, L.M. (2006) Bone sialoprotein mediates the tumor cell-targeted prometastatic activity of transforming growth factor beta in a mouse model of breast cancer. Cancer Research, 66, 6327-6335. http://dx.http://dx.doi.org/10.1158/0008-5472.CAN-06-0 068 [126] Nam, J.S., Terabe, M., Kang, M.J., Chae, H., Voong, N., Yang, Y.A., Laurence, A., Michalowska, A., Mamura, M., Lonning, S., Berzofsky, J.A. and Wakefield, L.M. (2008) Transforming growth factor beta subverts the immune system into directly promoting tumor growth through in- terleukin-17. Cancer Research, 68, 3915-3923. http://dx.http://dx.doi.org/10.1158/0008-5472.CAN-08-0 206 [127] Nam, J.S., Terabe, M., Mamura, M., Kang, M.J., Chae, H., Stuelten, C., Kohn, E., Tang, B., Sabzevari, H., Anver, M.R., Lawrence, S., Danielpour, D., Lonning, S., Berzof- sky, J.A. and Wakefield, L.M. (2008) An anti-transform- ing growth factor beta antibody suppresses metastasis via cooperative effects on multiple cell compartments. Can- cer Research, 68, 3835-3843. http://dx.http://dx.doi.org/10.1158/0008-5472.CAN-08-0 215 [128] Biswas, S., Nyman, J.S., Alvarez, J., Chakrabarti, A., Ayres, A., Sterling, J., Edwards, J., Rana, T., Johnson, R., Perrien, D.S., Lonning, S., Shyr, Y., Matrisian, L.M. and Mundy, G.R. (2011) Anti-Transforming Growth Factor ß Antibody Treatment Rescues Bone Loss and Prevents Breast Cancer Metastasis to Bone. PloS ONE, 6, e27090. http://dx.http://dx.doi.org/10.1371/journal.pone.0027090 [129] Bandyopadhyay, A., Zhu, Y., Cibull, M.L., Bao, L., Chen, C. and Sun, L. (1999) A soluble transforming growth factor beta type III receptor suppresses tumorigenicity and metastasis of human breast cancer MDA-MB-231 cells. Cancer Research, 59, 5041-5046. [130] Bandyopadhyay, A., Lopez-Casillas. F., Malik, S.N., Montiel, J.L., Mendoza, V., Yang, J. and Sun, L.Z. (2002) Antitumor activity of a recombinant soluble betaglycan in human breast cancer xenograft. Cancer Research, 62, 4690-4695. [131] Bennett, C.F. and Swayze, E.E. (2010) RNA targeting therapeutics: molecular mechanisms of antisense oligonu- cleotides as a therapeutic platform. Annual Review of Pharmacology and Toxicology, 50, 259-293. http://dx.http://dx.doi.org/10.1146/annurev.pharmtox.010 909.105654 [132] Crooke, S.T. (2004) Progress in antisense technology. Annual Review of Medicine, 55, 61-95. http://dx.http://dx.doi.org/10.1146/annurev.med.55.09190 2.104408 [133] Inman, G.J., Nicolas, F.J., Callahan, J.F., Harling, J.D., Gaster, L.M., Reith, A.D., Laping, N.J. and Hill, C.S. (2002) SB-431542 is a potent and specific inhibitor of transforming growth factor-beta superfamily type I acti- vin receptor-like kinase (ALK) receptors ALK4, ALK5, and ALK7. Molecular Pharmacology, 62, 65-74. http://dx.http://dx.doi.org/10.1124/mol.62.1.65 [134] Petersen, M., Thorikay, M., Deckers, M., Dinther, M., Grygielko, E.T., Gellibert, F., Gouville, A.C., Huet, S., Dijke, P. and Laping, N.J. (2008) Oral administration of Copyright © 2013 SciRes. OPEN ACCESS
 A. Chiechi et al. / Advances in Bioscience and Biotechnology 4 (2013) 15-30 Copyright © 2013 SciRes. 30 OPEN ACCESS GW788388, an inhibitor of TGF-beta type I and II recep- tor kinases, decreases renal fibrosis. Kidney International, 73, 705-715. http://dx.http://dx.doi.org/10.1038/sj.ki.5002717 [135] Tojo, M., Hamashima, Y., Hanyu, A., Kajimoto, T., Saitoh, M., Miyazono, K., Node, M. and Imamura, T. (2005) The ALK-5 inhibitor A-83-01 inhibits Smad sig- naling and epithelial-to-mesenchymal transition by trans- forming growth factor-beta. Cancer Science, 96, 791-800. http://dx.http://dx.doi.org/10.1111/j.1349-7006.2005.001 03.x [136] Byfield, S.D. and Roberts, A.B. (2004) Lateral signaling enhances TGF-beta response complexity. Trends in Cell Biology, 14, 107-111. http://dx.http://dx.doi.org/10.1016/j.tcb.2004.01.001 [137] Fu, K., Corbley, M.J., Sun, L., Friedman, J.E., Shan, F., Papadatos, J.L., Costa, D., Lutterodt, F., Sweigard, H., Bowes, S., Choi, M., Boriack-Sjodin, P.A., Arduini, R.M., Sun, D., Newman, M.N., Zhang, X., Mead, J.N., Chuaqui, C.E., Cheung, H.K., Cornebise, M., Carter, M.B., Josiah, S., Singh, J., Lee, W.C., Gill, A. and Ling, L.E. (2008) SM16, an orally active TGF-beta type I receptor inhibitor prevents myofibroblast induction and vascular fibrosis in the rat carotid injury model. Arteriosclerosis, Thrombosis, and Vascular Biology, 28, 665-671. http://dx.http://dx.doi.org/10.1161/ATVBAHA.107.1580 30 [138] Ehata, S., Hanyu, A., Fujime, M., Katsuno, Y,, Fukunaga, E., Goto, K., Ishikawa, Y., Nomura, K., Yokoo, H., Shi- mizu, T., Ogata, E., Miyazono, K., Shimizu, K. and Ima- mura, T. (2007) Ki26894, a novel transforming growth factor-beta type I receptor kinase inhibitor, inhibits in vitro invasion and in vivo bone metastasis of a human breast cancer cell line. Cancer Science, 98, 127-133. http://dx.http://dx.doi.org/10.1111/j.1349-7006.2006.003 57.x [139] Mohammad, K.S., Chen, C.G., Balooch, G., Stebbins, E., McKenna, C.R., Davis, H., Niewolna, M., Peng, X.H., Nguyen, D.H., Ionova-Martin, S.S., Bracey, J.W., Hogue, W.R., Wong, D.H., Ritchie, R.O., Suva, L.J., Derynck, R., Guise, T.A. and Alliston T. (2009) Pharmacologic inhibi- tion of the TGF-beta type I receptor kinase has anabolic and anti-catabolic effects on bone. PLoS ONE, 4, e5275. http://dx.http://dx.doi.org/10.1371/journal.pone.0005275 [140] Institute AAMCTCNC. (2009) Topical halofuginone hydrobromide in treating patients with HIV-related Ka- posi’s sarcoma. National Cancer Institute. http://clinicaltrials.gov/ct2/show/NCT00064142, [141] Juárez, P., Mohammad, K.S., Yin, J.J., Fournier, P.G., McKenna, R.C., Davis, H.W., Peng, X.H., Niewolna, M., Javelaud, D., Chirgwin, J.M., Mauviel, A. and Guise. T.A. (2012) Halofuginone inhibits the establishment and pro- gression of melanoma bone metastases. Cancer Research, 1, 6247-6256. http://dx.http://dx.doi.org/10.1158/0008-5472.CAN-12-1 444 [142] Gadir, N., Jackson, D.N., Lee, E. and Foster, D.A. (2008) Defective TGF-beta signaling sensitizes human cancer cells to rapamycin. Oncogene, 27, 1055-1062. http://dx.http://dx.doi.org/10.1038/sj.onc.1210721 [143] Filyak, Y., Filyak, O. and Stoika, R. (2007) Transforming growth factor beta-1 enhances cytotoxic effect of doxoru- bicin in human lung adenocarcinoma cells of A549 line. Cell Biology International, 31, 851-855. http://dx.http://dx.doi.org/10.1016/j.cellbi.2007.02.008 [144] Taniguchi, Y., Kawano, K., Minowa, T., Sugino, T., Shi- mojo, Y. and Maitani, Y. (2010) Enhanced antitumor ef- ficacy of folate-linked liposomal doxorubicin with TGF- beta type I receptor inhibitor. Cancer Science, 101, 2207- 2213. http://dx.http://dx.doi.org/10.1111/j.1349-7006.2010.016 46.x [145] Mohammad, K.S., Stebbins, E.G., Kingsley, L., Fournier, P.G.J., Niewolna, M., McKenna, C.R., Peng, X., Higgins, L., Wong, D. and Guise, T.A. (2008) Combined trans- forming growth factor b receptor I kinase inhibitor and biphosphonates are additve to reduce breast cancer bone metastases. Journal of Bone and Mineral Research, 23, F275. [146] Hengst, V., Oussoren, C., Kissel, T. and Storm, G. (2007) Bone targeting potential of bisphosphonate-targeted lipo- somes. Preparation, characterization and hydroxyapatite binding in vitro. International Journal of Pharmaceutics, 331, 224-227. http://dx.http://dx.doi.org/10.1016/j.ijpharm.2006.11.024
|