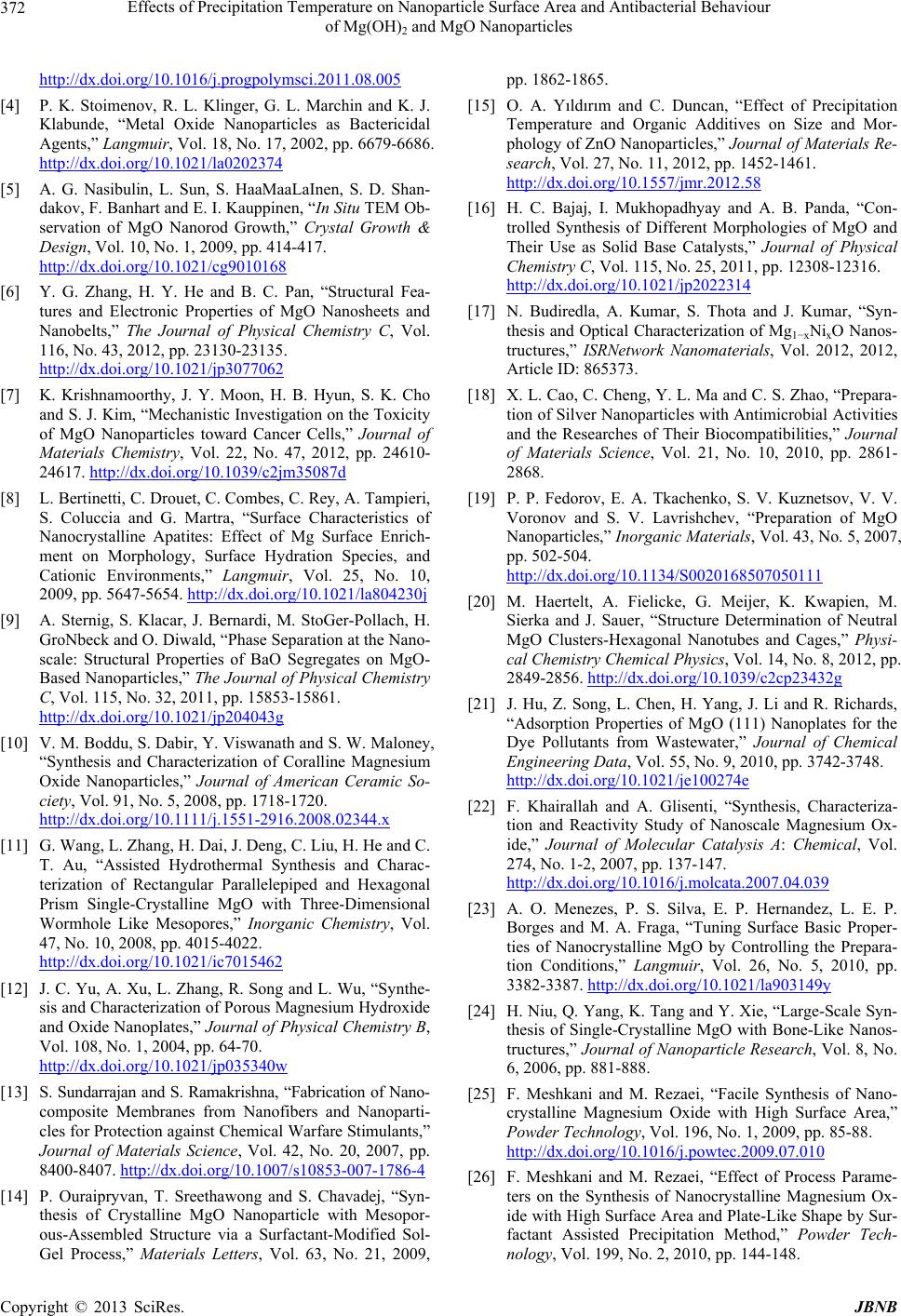
Effects of Precipitation Temperature on Nanoparticle Surface Area and Antibacterial Behaviour
of Mg(OH)2 and MgO Nanoparticles
372
http://dx.doi.org/10.1016/j.progpolymsci.2011.08.005
[4] P. K. Stoimenov, R. L. Klinger, G. L. Marchin and K. J.
Klabunde, “Metal Oxide Nanoparticles as Bactericidal
Agents,” Langmuir, Vol. 18, No. 17, 2002, pp. 6679-6686.
http://dx.doi.org/10.1021/la0202374
[5] A. G. Nasibulin, L. Sun, S. HaaMaaLaInen, S. D. Shan-
dakov, F. Banhart and E. I. Kauppinen, “In Situ TEM Ob-
servation of MgO Nanorod Growth,” Crystal Growth &
Design, Vol. 10, No. 1, 2009, pp. 414-417.
http://dx.doi.org/10.1021/cg9010168
[6] Y. G. Zhang, H. Y. He and B. C. Pan, “Structural Fea-
tures and Electronic Properties of MgO Nanosheets and
Nanobelts,” The Journal of Physical Chemistry C, Vol.
116, No. 43, 2012, pp. 23130-23135.
http://dx.doi.org/10.1021/jp3077062
[7] K. Krishnamoorthy, J. Y. Moon, H. B. Hyun, S. K. Cho
and S. J. Kim, “Mechanistic Investigation on the Toxicity
of MgO Nanoparticles toward Cancer Cells,” Journal of
Materials Chemistry, Vol. 22, No. 47, 2012, pp. 24610-
24617. http://dx.doi.org/10.1039/c2jm35087d
[8] L. Bertinetti, C. Drouet, C. Combes, C. Rey, A. Tampieri,
S. Coluccia and G. Martra, “Surface Characteristics of
Nanocrystalline Apatites: Effect of Mg Surface Enrich-
ment on Morphology, Surface Hydration Species, and
Cationic Environments,” Langmuir, Vol. 25, No. 10,
2009, pp. 5647-5654. http://dx.doi.org/10.1021/la804230j
[9] A. Sternig, S. Klacar, J. Bernardi, M. StoGer-Pollach, H.
GroNbeck and O. Diwald, “Phase Separation at the Nano-
scale: Structural Properties of BaO Segregates on MgO-
Based Nanoparticles,” The Journal of Physical Chemistry
C, Vol. 115, No. 32, 2011, pp. 15853-15861.
http://dx.doi.org/10.1021/jp204043g
[10] V. M. Boddu, S. Dabir, Y. Viswanath and S. W. Maloney,
“Synthesis and Characterization of Coralline Magnesium
Oxide Nanoparticles,” Journal of American Ceramic So-
ciety, Vol. 91, No. 5, 2008, pp. 1718-1720.
http://dx.doi.org/10.1111/j.1551-2916.2008.02344.x
[11] G. Wang, L. Zhang, H. Dai, J. Deng, C. Liu, H. He and C.
T. Au, “Assisted Hydrothermal Synthesis and Charac-
terization of Rectangular Parallelepiped and Hexagonal
Prism Single-Crystalline MgO with Three-Dimensional
Wormhole Like Mesopores,” Inorganic Chemistry, Vol.
47, No. 10, 2008, pp. 4015-4022.
http://dx.doi.org/10.1021/ic7015462
[12] J. C. Yu, A. Xu, L. Zhang, R. Song and L. Wu, “Synthe-
sis and Characterization of Porous Magnesium Hydroxide
and Oxide Nanoplates,” Journal of Physical Chemistry B,
Vol. 108, No. 1, 2004, pp. 64-70.
http://dx.doi.org/10.1021/jp035340w
[13] S. Sundarrajan and S. Ramakrishna, “Fabrication of Nano-
composite Membranes from Nanofibers and Nanoparti-
cles for Protection against Chemical Warfare Stimulants,”
Journal of Materials Science, Vol. 42, No. 20, 2007, pp.
8400-8407. http://dx.doi.org/10.1007/s10853-007-1786-4
[14] P. Ouraipryvan, T. Sreethawong and S. Chavadej, “Syn-
thesis of Crystalline MgO Nanoparticle with Mesopor-
ous-Assembled Structure via a Surfactant-Modified Sol-
Gel Process,” Materials Letters, Vol. 63, No. 21, 2009,
pp. 1862-1865.
[15] O. A. Yıldırım and C. Duncan, “Effect of Precipitation
Temperature and Organic Additives on Size and Mor-
phology of ZnO Nanoparticles,” Journal of Materials Re-
search, Vol. 27, No. 11, 2012, pp. 1452-1461.
http://dx.doi.org/10.1557/jmr.2012.58
[16] H. C. Bajaj, I. Mukhopadhyay and A. B. Panda, “Con-
trolled Synthesis of Different Morphologies of MgO and
Their Use as Solid Base Catalysts,” Journal of Physical
Chemistry C, Vol. 115, No. 25, 2011, pp. 12308-12316.
http://dx.doi.org/10.1021/jp2022314
[17] N. Budiredla, A. Kumar, S. Thota and J. Kumar, “Syn-
thesis and Optical Characterization of Mg1−xNixO Nanos-
tructures,” ISRNetwork Nanomaterials, Vol. 2012, 2012,
Article ID: 865373.
[18] X. L. Cao, C. Cheng, Y. L. Ma and C. S. Zhao, “Prepara-
tion of Silver Nanoparticles with Antimicrobial Activities
and the Researches of Their Biocompatibilities,” Journal
of Materials Science, Vol. 21, No. 10, 2010, pp. 2861-
2868.
[19] P. P. Fedorov, E. A. Tkachenko, S. V. Kuznetsov, V. V.
Voronov and S. V. Lavrishchev, “Preparation of MgO
Nanoparticles,” Inorganic Materials, Vol. 43, No. 5, 2007,
pp. 502-504.
http://dx.doi.org/10.1134/S0020168507050111
[20] M. Haertelt, A. Fielicke, G. Meijer, K. Kwapien, M.
Sierka and J. Sauer, “Structure Determination of Neutral
MgO Clusters-Hexagonal Nanotubes and Cages,” Physi-
cal Chemistry Chemical Physics, Vol. 14, No. 8, 2012, pp.
2849-2856. http://dx.doi.org/10.1039/c2cp23432g
[21] J. Hu, Z. Song, L. Chen, H. Yang, J. Li and R. Richards,
“Adsorption Properties of MgO (111) Nanoplates for the
Dye Pollutants from Wastewater,” Journal of Chemical
Engineering Data, Vol. 55, No. 9, 2010, pp. 3742-3748.
http://dx.doi.org/10.1021/je100274e
[22] F. Khairallah and A. Glisenti, “Synthesis, Characteriza-
tion and Reactivity Study of Nanoscale Magnesium Ox-
ide,” Journal of Molecular Catalysis A: Chemical, Vol.
274, No. 1-2, 2007, pp. 137-147.
http://dx.doi.org/10.1016/j.molcata.2007.04.039
[23] A. O. Menezes, P. S. Silva, E. P. Hernandez, L. E. P.
Borges and M. A. Fraga, “Tuning Surface Basic Proper-
ties of Nanocrystalline MgO by Controlling the Prepara-
tion Conditions,” Langmuir, Vol. 26, No. 5, 2010, pp.
3382-3387. http://dx.doi.org/10.1021/la903149y
[24] H. Niu, Q. Yang, K. Tang and Y. Xie, “Large-Scale Syn-
thesis of Single-Crystalline MgO with Bone-Like Nanos-
tructures,” Journal of Nanoparticle Research, Vol. 8, No.
6, 2006, pp. 881-888.
[25] F. Meshkani and M. Rezaei, “Facile Synthesis of Nano-
crystalline Magnesium Oxide with High Surface Area,”
Powder Technology, Vol. 196, No. 1, 2009, pp. 85-88.
http://dx.doi.org/10.1016/j.powtec.2009.07.010
[26] F. Meshkani and M. Rezaei, “Effect of Process Parame-
ters on the Synthesis of Nanocrystalline Magnesium Ox-
ide with High Surface Area and Plate-Like Shape by Sur-
factant Assisted Precipitation Method,” Powder Tech-
nology, Vol. 199, No. 2, 2010, pp. 144-148.
Copyright © 2013 SciRes. JBNB