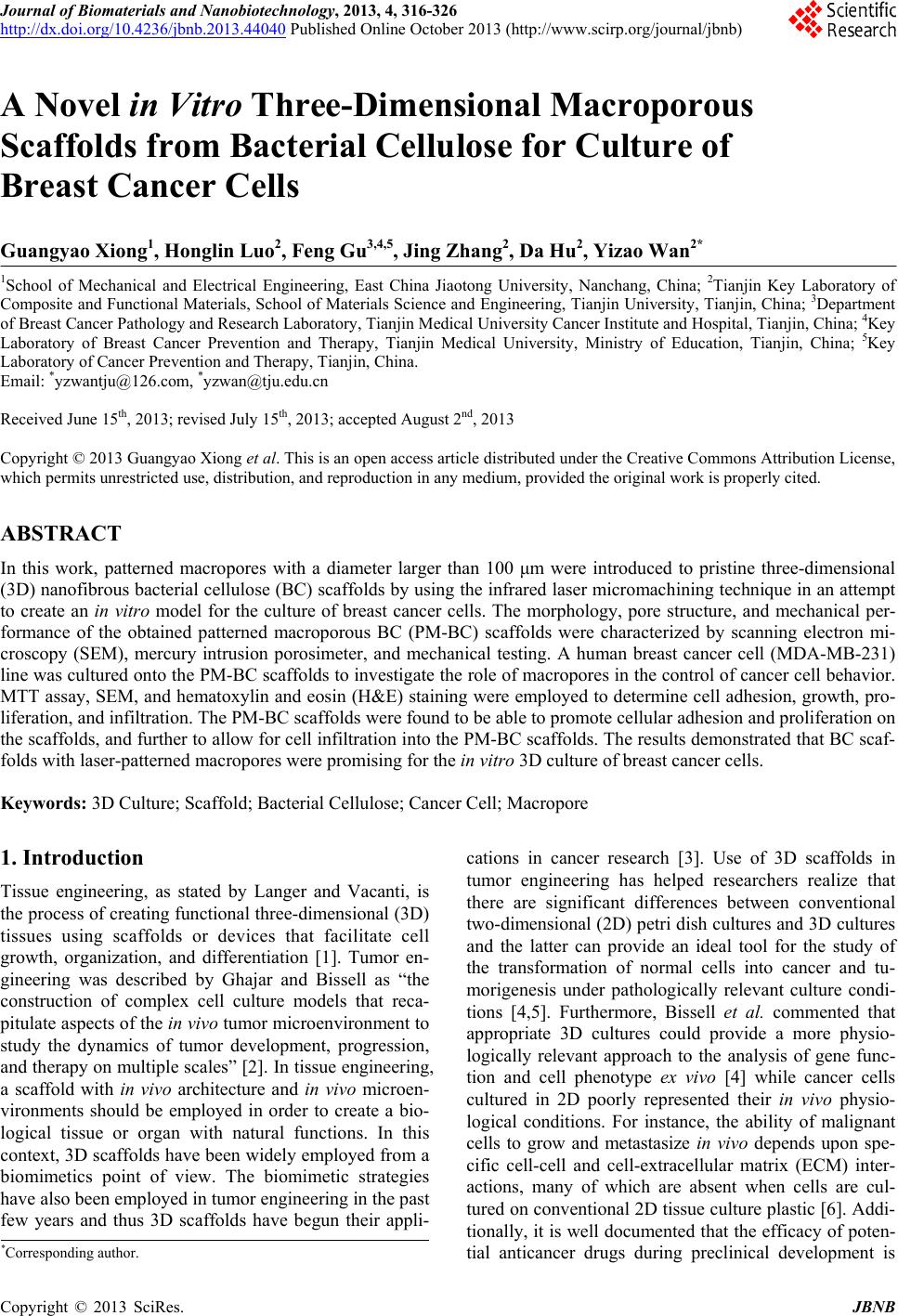 Journal of Biomaterials and Nanobiotechnology, 2013, 4, 316-326 http://dx.doi.org/10.4236/jbnb.2013.44040 Published Online October 2013 (http://www.scirp.org/journal/jbnb) A Novel in Vitro Three-Dimensional Macroporous Scaffolds from Bacterial Cellulose for Culture of Breast Cancer Cells Guangyao Xiong1, Honglin Luo2, Feng Gu3,4,5, Jing Zhang2, Da Hu2, Yizao Wan2* 1School of Mechanical and Electrical Engineering, East China Jiaotong University, Nanchang, China; 2Tianjin Key Laboratory of Composite and Functional Materials, School of Materials Science and Engineering, Tianjin University, Tianjin, China; 3Department of Breast Cancer Pathology and Research Laboratory, Tianjin Medical University Cancer Institute and Hospital, Tianjin, China; 4Key Laboratory of Breast Cancer Prevention and Therapy, Tianjin Medical University, Ministry of Education, Tianjin, China; 5Key Laboratory of Cancer Prevention and Therapy, Tianjin, China. Email: *yzwantju@126.com, *yzwan@tju.edu.cn Received June 15th, 2013; revised July 15th, 2013; accepted August 2nd, 2013 Copyright © 2013 Guangyao Xiong et al. This is an open access article distributed under the Creative Commons Attribution License, which permits unrestricted use, distribution, and reproduction in any medium, provided the original work is properly cited. ABSTRACT In this work, patterned macropores with a diameter larger than 100 μm were introduced to pristine three-dimensional (3D) nanofibrous bacterial cellulose (BC) scaffolds by using the infrared laser micromachining technique in an attempt to create an in vitro model for the culture of breast cancer cells. The morphology, pore structure, and mechanical per- formance of the obtained patterned macroporous BC (PM-BC) scaffolds were characterized by scanning electron mi- croscopy (SEM), mercury intrusion porosimeter, and mechanical testing. A human breast cancer cell (MDA-MB-231) line was cultured onto the PM-BC scaffolds to investigate the role of macropores in the control of cancer cell behavior. MTT assay, SEM, and hematoxylin and eosin (H&E) staining were employed to determine cell adhesion, growth, pro- liferation, and infiltration. The PM-BC scaffolds were found to be able to promote cellular adhesion and proliferation on the scaffolds, and further to allow for cell infiltration into the PM-BC scaffolds. The results demonstrated that BC scaf- folds with laser-patterned macropores were promising for the in vitro 3D culture of breast cancer cells. Keywords: 3D Culture; Scaffold; Bacterial Cellulose; Cancer Cell; Macropore 1. Introduction Tissue engineering, as stated by Langer and Vacanti, is the process of creating functional three-dimensional (3D) tissues using scaffolds or devices that facilitate cell growth, organization, and differentiation [1]. Tumor en- gineering was described by Ghajar and Bissell as “the construction of complex cell culture models that reca- pitulate aspects of the in vivo tumor microenvironment to study the dynamics of tumor development, progression, and therapy on multiple scales” [2]. In tissue engineering, a scaffold with in vivo architecture and in vivo microen- vironments should be employed in order to create a bio- logical tissue or organ with natural functions. In this context, 3D scaffolds have been widely employed from a biomimetics point of view. The biomimetic strategies have also been employed in tumor engineering in the past few years and thus 3D scaffolds have begun their appli- cations in cancer research [3]. Use of 3D scaffolds in tumor engineering has helped researchers realize that there are significant differences between conventional two-dimensional (2D) petri dish cultures and 3D cultures and the latter can provide an ideal tool for the study of the transformation of normal cells into cancer and tu- morigenesis under pathologically relevant culture condi- tions [4,5]. Furthermore, Bissell et al. commented that appropriate 3D cultures could provide a more physio- logically relevant approach to the analysis of gene func- tion and cell phenotype ex vivo [4] while cancer cells cultured in 2D poorly represented their in vivo physio- logical conditions. For instance, the ability of malignant cells to grow and metastasize in vivo depends upon spe- cific cell-cell and cell-extracellular matrix (ECM) inter- actions, many of which are absent when cells are cul- tured on conventional 2D tissue culture plastic [6]. Addi- tionally, it is well documented that the efficacy of poten- tial anticancer drugs during preclinical development is *Corresponding author. Copyright © 2013 SciRes. JBNB
 A Novel in Vitro Three-Dimensional Macroporous Scaffolds from Bacterial Cellulose for Culture of Breast Cancer Cells 317 generally tested in vitro using cancer cells grown in scaf- folds. Conventional evaluation is based upon the 2D cul- ture; however, a significant discrepancy in their efficacy is observed when these drugs are evaluated in vivo. For instance, Horning et al. declared that the cells grown in 3D scaffolds are more resistant to chemotherapy than those grown in 2D culture [7]. Therefore, creating 3D culture which mimics in vivo conditions is of primary importance to the evaluation of the efficacy of anticancer drugs as well as the study of tumor biology [8]. To date, various biomaterials including natural and synthetic polymers have been used to fabricate 3D scaf- folds for cancer research and anticancer drugs tests. Col- lagen has been widely utilized to culture cells owing to its excellent characteristics, including biocompatibility, mechanical strength, degradability and limited immuno- genicity [9,10]. Other biomaterials such as chitosanalgi- nate [11], polyacrylamide [12], poly (lactic acid) (PLA) [7,13], poly(lactic-co-glycolide) (PLGA) [7,13], hyalu- ronan [14], and silk fibroin protein [15] have been ex- plored as 3D models for cancer research. Over the last two decades, various techniques have been developed to fabricate biomimetic scaffolds for tis- sue engineering and later for tumor engineering. Elec- trospinning has been the most widely used technique to create 3D scaffolds composed of nanofibers or mostly sub-microfibers [16,17]. Electrospinning is a relatively simple and scalable nanotechnological method for the generation of nanostructured scaffolds that closely mimic the dimensions of collagen fibrils of ECM and a very recent report showed the capability of creating 3D scaf- folds [18]. However, electrospun fibers do not allow pro- per infiltration of the cells to the core of the scaffolds due to the limited pore size. Another substantial disadvantage is that the diameters of the fibers are usually at the upper limits of the 50 - 500 nm range seen in natural ECM [19]. Therefore, scaffolds which allow effective cell seeding and penetration, have controllable fiber diameter in nano- scale and sufficient material properties are highly desir- able in both tissue engineering and tumor engineering. These requirements and the tremendous interest in ex- ploring the potency of biomimetic scaffolds have urged researchers to continuously develop more techniques. Molecular self assembly [20] and phase separation [21, 22] have emerged as other promising techniques for the fabrication of 3D nanofibrous scaffolds. Efforts are still being made to find more alternative methodologies so as to simultaneously control morphological, mechanical, and chemical performances of scaffolds. It is noteworthy that bacterial cellulose (BC), a natural nanofibrous polymer, has attracted more and more atten- tion. BC is not fabricated by electrospinning; instead it is synthesized extracellularly by the bacterium Acetobacter xylinum. BC has received enormous research interest and the number of reports on BC has showed a tremendous increase over recent years (reviewed by Petersen and Gatenholm [23], and by Klemm and colleagues [24]). BC nanofibers can have a diameter as small as 10 nm which is the low limit of natural ECM fibers ranging from 10 to several hundreds of nanometers [19]. BC is superior to plant cellulose (PC) owing to its high purity, 3D mor- phology, high crystallinity, high tensile strength and modulus (the effective modulus of single fibrils of BC ranged from 79 to 88 GPa versus 29 to 36 GPa for plant cellulose [25]) in combination with a variety of proper- ties such as high water holding capacity, large surface area, and particularly good biocompatibility. For instance, a long-term biocompatibility study conducted by Pertile et al. confirmed that BC caused a mild and benign in- flammatory reaction that decreased along time and did not elicit a foreign body reaction [26]. Very recently, a long-term study on in vivo biocompatibility of BC has been reported and BC was defined as a biocompatible material [27]. A latest study by Favi et al. showed that BC scaffolds were cytocompatible and could support cellular adhesion and proliferation, and allowed for os- teogenic and chondrogenic differentiation of equine- derived bone marrow mesenchymal stem cells (EqMSCs) [28]. Shi and co-workers declared that BC was a good localized delivery system for bone morphogenetic pro- tein-2 (BMP) and would be a potential candidate in bone tissue engineering [29]. Another study by Saska et al. found that BC membranes functionalized with osteogenic growth peptide (OGP) and its C-terminal pentapeptide OGP could be used in bone tissue engineering/regenera- tion [30]. Very recently, BC microstrands were fabricated which could serve as a pathway of nutrition and oxygen to feed the cells in the central region of a macroscopic tissue [31]. Though extensive research has been carried out to determine the potential of BC as tissue engineering scaffolds, investigation on BC scaffolds for tumor engi- neering has been very limited. The only pioneering study on the in vitro culture of cancer cells including the hu- man androgen-independent prostate cancer cell line (PC- 3), murine renal cancer cell line (RENCA), and human breast cancer cell line (MDA-MB-231) on BC scaffolds demonstrated that these cancer cells cultured on BC did not have observable protrusions indicating undesirable cancer cell responses, which were ascribed to the absence of manufactured large porosity [32]. It has been accepted that pore structure is an essential consideration in the development of scaffolds for tissue engineering and pores must be interconnected and large enough to allow for cell growth, migration and nutrient flow, and for vascularization, new tissue formation and remodeling so as to facilitate host tissue integration upon Copyright © 2013 SciRes. JBNB
 A Novel in Vitro Three-Dimensional Macroporous Scaffolds from Bacterial Cellulose for Culture of Breast Cancer Cells Copyright © 2013 SciRes. JBNB 318 2.2. Preparation of BC Pellicles implantation. Furthermore, pore volume (porosity), shape and distribution also should be considered [33]. A recent study demonstrated that the acrylate copolymers-based scaffolds with aligned channels achieved a uniform colo- nization by neural cells [34]. Our previous work indi- cated that the BC/Gelatin/Hydroxyapatite scaffolds with patterned pores supported the attachment and prolifera- tion of chondrogenic rat cell [35]. However, how the aligned pore channels affect cancer cell behavior has not been reported. The preparation and purification procedures of BC pelli- cles were described previously [37,38]. Briefly, the bac- terial strain, Acetobacter xylinum X-2, was grown in the culture media containing 0.3 wt% green tea powder (analytical grade) and 5 wt% sucrose (analytical grade) for 7 days. The pH of the medium was adjusted to 4.5 by acetic acid. BC pellicles were purified by soaking in de- ionized water at 90˚C for 2 h followed by boiling in a 0.5 M NaOH solution for 15 min. The BC pellicles with 9 cm in diameter were then washed with deionized water several times and soaked in 1% NaOH for 2 days. Finally, the BC pellicles were washed free of alkali. Therefore, the purpose of the present study was, for the first time, to determine the feasibility of the BC scaf- folds with aligned channels as effective in vitro cancer models. To this end, the BC scaffolds with patterned ma- cropores (with a diameter greater than 100 μm according to literatures [33,36]) were fabricated by an infrared laser micromachining technique and a human breast cancer cell line (MDA-MB-231), as a model cancer cell line, was seeded onto the 3D macroporous BC scaffolds to investigate the cancer cellular responses to the scaffolds. The cell behavior on these 3D macroporous BC scaffolds was compared with that on the pristine BC scaffolds [32]. 2.3. Preparation of 3D BC Scaffolds with Patterned Macropores via Laser Technology Many previous studies reported the creation of patterned pores in solid materials such as titanium [39,40], and biodegradable polymers [41-43] mostly by using ultra- violet (UV) or femtosecond laser micromachining. In this study, patterned pores in BC hydrogels were created by using infrared laser. Figure 1 illustrates the laser-micro- machining process. The predetermined patterns (includ- ing distance between neighboring pores and pore diame- ter) were initially designed by a commercial CAD soft- ware and input to a computer. The BC hydrogels were then perforated using a CO2 excimer laser (wavelength 10.6 μm) according to the designed patterns to obtain BC scaffolds with patterned macropores (named as PM-BC hereinafter). The laser generated a stable power of 80 W, the exposure time was about 1 - 2 s for every pore de- pending on the thickness of the BC samples and the pore diameter could be altered by adjusting the distance be- tween specimens and laser focus (i.e. parameter d in Figure 1). In this work, three PM-BC scaffolds with dif- ferent pore size and pore density were fabricated and 2. Materials and Methods 2.1. Materials The reagents used in this work included glucose, peptone, yeast extract, disdium phosphate and acetate acid (Acros, Biochemical), deionized water (Aqoapro CO., Ltd, Chong- qing, China), sodium hydroxide, ethyl alcohol, formal- dehyde, glutaraldehyde, sodium chloride (Tianjin Tianda Tianlong Sci. & Tech. Co., Ltd., Tianjin, China), hank’s balanced salt solution (HBSS), phosphate buffered saline (PBS), dimethyl sulfoxide (DMSO, Gibco), and gelatin (Sigma, analytical grade). All chemicals were used as received without further purification. Figure 1. Schematic setup of the laser-patterning system used in this work (the parameter d refers to the distance from laser focus to the specimen).
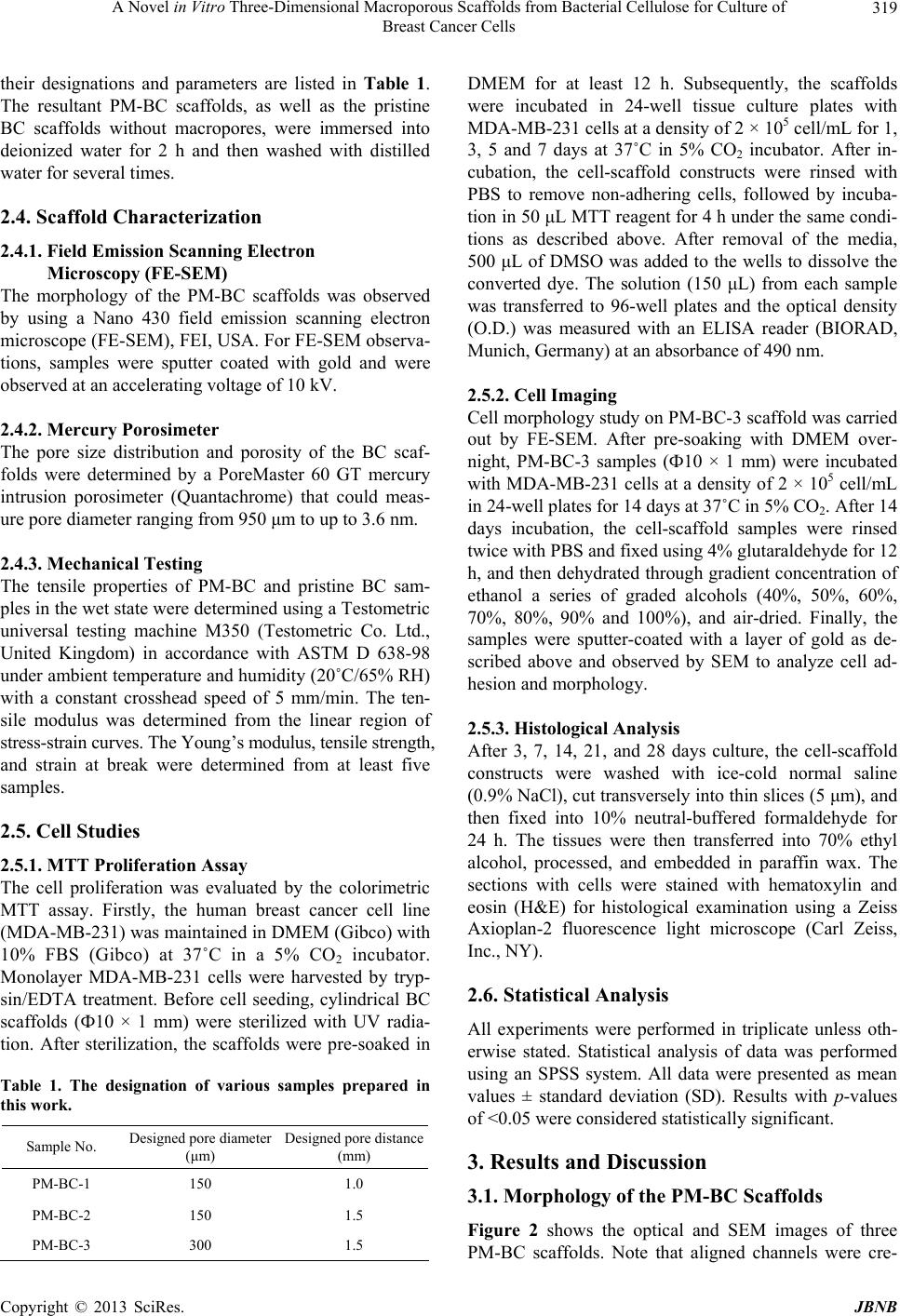 A Novel in Vitro Three-Dimensional Macroporous Scaffolds from Bacterial Cellulose for Culture of Breast Cancer Cells 319 their designations and parameters are listed in Table 1. The resultant PM-BC scaffolds, as well as the pristine BC scaffolds without macropores, were immersed into deionized water for 2 h and then washed with distilled water for several times. 2.4. Scaffold Characterization 2.4.1. Field Emission Scanning Electron Microscopy (F E -SEM ) The morphology of the PM-BC scaffolds was observed by using a Nano 430 field emission scanning electron microscope (FE-SEM), FEI, USA. For FE-SEM observa- tions, samples were sputter coated with gold and were observed at an accelerating voltage of 10 kV. 2.4.2. Mercury Porosimeter The pore size distribution and porosity of the BC scaf- folds were determined by a PoreMaster 60 GT mercury intrusion porosimeter (Quantachrome) that could meas- ure pore diameter ranging from 950 μm to up to 3.6 nm. 2.4.3. Mechanical Te sting The tensile properties of PM-BC and pristine BC sam- ples in the wet state were determined using a Testometric universal testing machine M350 (Testometric Co. Ltd., United Kingdom) in accordance with ASTM D 638-98 under ambient temperature and humidity (20˚C/65% RH) with a constant crosshead speed of 5 mm/min. The ten- sile modulus was determined from the linear region of stress-strain curves. The Young’s modulus, tensile strength, and strain at break were determined from at least five samples. 2.5. Cell Studies 2.5.1. MTT Proliferation Assay The cell proliferation was evaluated by the colorimetric MTT assay. Firstly, the human breast cancer cell line (MDA-MB-231) was maintained in DMEM (Gibco) with 10% FBS (Gibco) at 37˚C in a 5% CO2 incubator. Monolayer MDA-MB-231 cells were harvested by tryp- sin/EDTA treatment. Before cell seeding, cylindrical BC scaffolds (Ф10 × 1 mm) were sterilized with UV radia- tion. After sterilization, the scaffolds were pre-soaked in Table 1. The designation of various samples prepared in this work. Sample No. Designed pore diameter (μm) Designed pore distance (mm) PM-BC-1 150 1.0 PM-BC-2 150 1.5 PM-BC-3 300 1.5 DMEM for at least 12 h. Subsequently, the scaffolds were incubated in 24-well tissue culture plates with MDA-MB-231 cells at a density of 2 × 105 cell/mL for 1, 3, 5 and 7 days at 37˚C in 5% CO2 incubator. After in- cubation, the cell-scaffold constructs were rinsed with PBS to remove non-adhering cells, followed by incuba- tion in 50 μL MTT reagent for 4 h under the same condi- tions as described above. After removal of the media, 500 μL of DMSO was added to the wells to dissolve the converted dye. The solution (150 μL) from each sample was transferred to 96-well plates and the optical density (O.D.) was measured with an ELISA reader (BIORAD, Munich, Germany) at an absorbance of 490 nm. 2.5.2. Cel l Imaging Cell morphology study on PM-BC-3 scaffold was carried out by FE-SEM. After pre-soaking with DMEM over- night, PM-BC-3 samples (Ф10 × 1 mm) were incubated with MDA-MB-231 cells at a density of 2 × 105 cell/mL in 24-well plates for 14 days at 37˚C in 5% CO2. After 14 days incubation, the cell-scaffold samples were rinsed twice with PBS and fixed using 4% glutaraldehyde for 12 h, and then dehydrated through gradient concentration of ethanol a series of graded alcohols (40%, 50%, 60%, 70%, 80%, 90% and 100%), and air-dried. Finally, the samples were sputter-coated with a layer of gold as de- scribed above and observed by SEM to analyze cell ad- hesion and morphology. 2.5.3. Hist ol o g i cal Anal ysis After 3, 7, 14, 21, and 28 days culture, the cell-scaffold constructs were washed with ice-cold normal saline (0.9% NaCl), cut transversely into thin slices (5 μm), and then fixed into 10% neutral-buffered formaldehyde for 24 h. The tissues were then transferred into 70% ethyl alcohol, processed, and embedded in paraffin wax. The sections with cells were stained with hematoxylin and eosin (H&E) for histological examination using a Zeiss Axioplan-2 fluorescence light microscope (Carl Zeiss, Inc., NY). 2.6. Statistical Analysis All experiments were performed in triplicate unless oth- erwise stated. Statistical analysis of data was performed using an SPSS system. All data were presented as mean values ± standard deviation (SD). Results with p-values of <0.05 were considered statistically significant. 3. Results and Discussion 3.1. Morphology of the PM-BC Scaffolds Figure 2 shows the optical and SEM images of three PM-BC scaffolds. Note that aligned channels were cre- Copyright © 2013 SciRes. JBNB
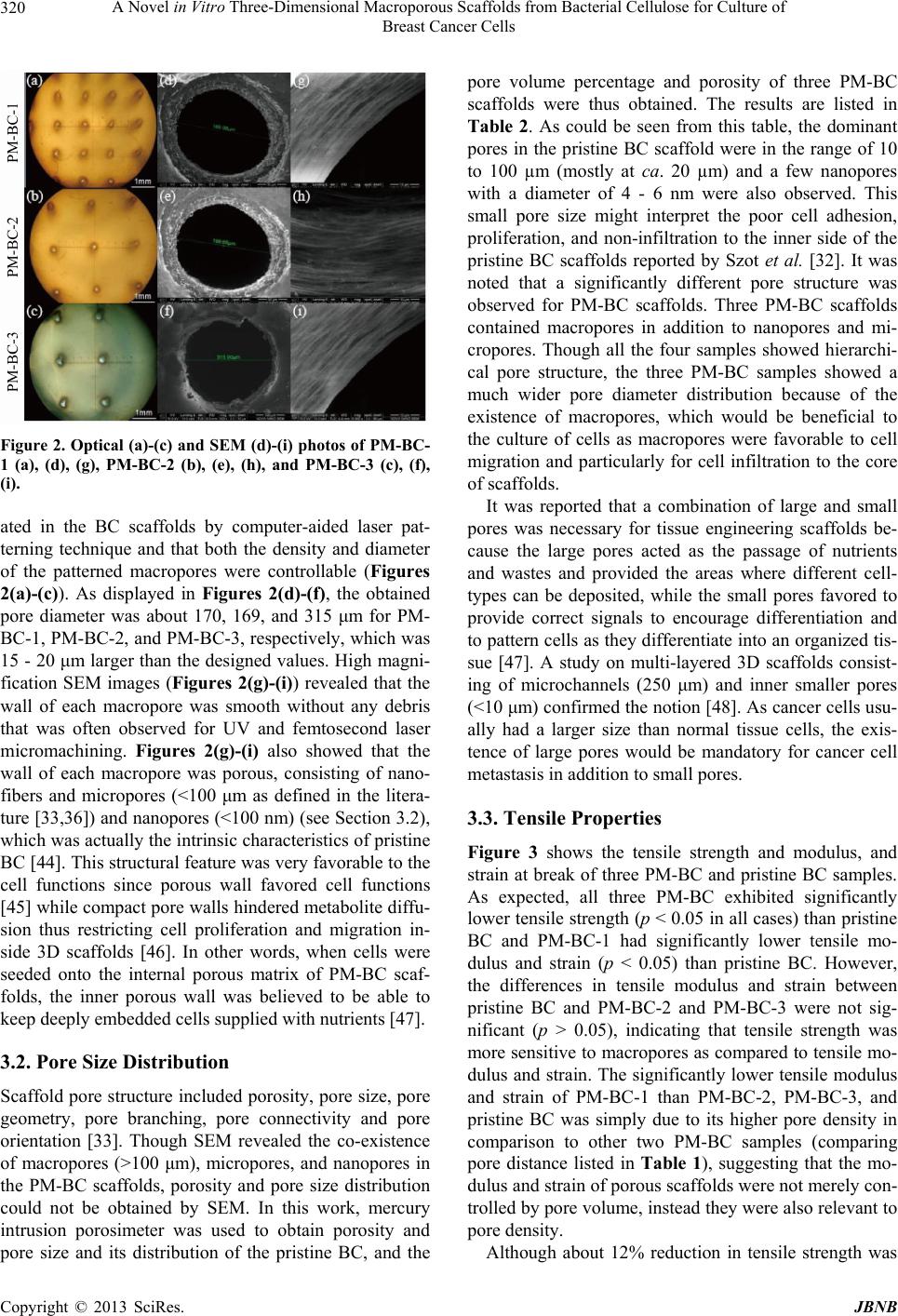 A Novel in Vitro Three-Dimensional Macroporous Scaffolds from Bacterial Cellulose for Culture of Breast Cancer Cells 320 Figure 2. Optical (a)-(c) and SEM (d)-(i) photos of PM-BC- 1 (a), (d), (g), PM-BC-2 (b), (e), (h), and PM-BC-3 (c), (f), (i). ated in the BC scaffolds by computer-aided laser pat- terning technique and that both the density and diameter of the patterned macropores were controllable (Figures 2(a)-(c)). As displayed in Figures 2(d)-(f), the obtained pore diameter was about 170, 169, and 315 μm for PM- BC-1, PM-BC-2, and PM-BC-3, respectively, which was 15 - 20 μm larger than the designed values. High magni- fication SEM images (Figures 2(g)-(i)) revealed that the wall of each macropore was smooth without any debris that was often observed for UV and femtosecond laser micromachining. Figures 2(g)-(i) also showed that the wall of each macropore was porous, consisting of nano- fibers and micropores (<100 μm as defined in the litera- ture [33,36]) and nanopores (<100 nm) (see Section 3.2), which was actually the intrinsic characteristics of pristine BC [44]. This structural feature was very favorable to the cell functions since porous wall favored cell functions [45] while compact pore walls hindered metabolite diffu- sion thus restricting cell proliferation and migration in- side 3D scaffolds [46]. In other words, when cells were seeded onto the internal porous matrix of PM-BC scaf- folds, the inner porous wall was believed to be able to keep deeply embedded cells supplied with nutrients [47]. 3.2. Pore Size Distribution Scaffold pore structure included porosity, pore size, pore geometry, pore branching, pore connectivity and pore orientation [33]. Though SEM revealed the co-existence of macropores (>100 μm), micropores, and nanopores in the PM-BC scaffolds, porosity and pore size distribution could not be obtained by SEM. In this work, mercury intrusion porosimeter was used to obtain porosity and pore size and its distribution of the pristine BC, and the pore volume percentage and porosity of three PM-BC scaffolds were thus obtained. The results are listed in Table 2. As could be seen from this table, the dominant pores in the pristine BC scaffold were in the range of 10 to 100 µm (mostly at ca. 20 µm) and a few nanopores with a diameter of 4 - 6 nm were also observed. This small pore size might interpret the poor cell adhesion, proliferation, and non-infiltration to the inner side of the pristine BC scaffolds reported by Szot et al. [32]. It was noted that a significantly different pore structure was observed for PM-BC scaffolds. Three PM-BC scaffolds contained macropores in addition to nanopores and mi- cropores. Though all the four samples showed hierarchi- cal pore structure, the three PM-BC samples showed a much wider pore diameter distribution because of the existence of macropores, which would be beneficial to the culture of cells as macropores were favorable to cell migration and particularly for cell infiltration to the core of scaffolds. It was reported that a combination of large and small pores was necessary for tissue engineering scaffolds be- cause the large pores acted as the passage of nutrients and wastes and provided the areas where different cell- types can be deposited, while the small pores favored to provide correct signals to encourage differentiation and to pattern cells as they differentiate into an organized tis- sue [47]. A study on multi-layered 3D scaffolds consist- ing of microchannels (250 μm) and inner smaller pores (<10 μm) confirmed the notion [48]. As cancer cells usu- ally had a larger size than normal tissue cells, the exis- tence of large pores would be mandatory for cancer cell metastasis in addition to small pores. 3.3. Tensile Properties Figure 3 shows the tensile strength and modulus, and strain at break of three PM-BC and pristine BC samples. As expected, all three PM-BC exhibited significantly lower tensile strength (p < 0.05 in all cases) than pristine BC and PM-BC-1 had significantly lower tensile mo- dulus and strain (p < 0.05) than pristine BC. However, the differences in tensile modulus and strain between pristine BC and PM-BC-2 and PM-BC-3 were not sig- nificant (p > 0.05), indicating that tensile strength was more sensitive to macropores as compared to tensile mo- dulus and strain. The significantly lower tensile modulus and strain of PM-BC-1 than PM-BC-2, PM-BC-3, and pristine BC was simply due to its higher pore density in comparison to other two PM-BC samples (comparing pore distance listed in Table 1), suggesting that the mo- dulus and strain of porous scaffolds were not merely con- trolled by pore volume, instead they were also relevant to pore density. Although about 12% reduction in tensile strength was Copyright © 2013 SciRes. JBNB
 A Novel in Vitro Three-Dimensional Macroporous Scaffolds from Bacterial Cellulose for Culture of Breast Cancer Cells Copyright © 2013 SciRes. JBNB 321 Table 2. Comparisons of pore volume percentage (%) and porosity (%) of various BC scaffolds. Micropores (%) Samples Nanopores (%) <100 nm 1 - 10 μm 10 - 100 μm Macropores (%) >100 μm Porosity (%) Pristine BC 1.36 25.19 66.81 0.00 92.05 PM-BC-1 1.33 23.88 65.95 1.28 92.16 PM-BC-2 1.30 24.65 65.38 2.14 92.23 PM-BC-3 1.30 24.08 63.88 4.38 92.44 folds still very competitive for tissue engineering and tumor engineering scaffolds in terms of mechanical per- formances. Pristine BCPM-BC-1PM-BC-2PM-BC-3 0.0 0.2 0.4 0.6 Tensile strength (MPa) (a) + Many previous studies on bone tissue engineering have suggested a need for pore size >300 µm for bone formation and vascularisation [40,45,50]. Considering cancer cells are usually larger in size than normal cells, PM-BC-3 with 315 µm pore size was believed to be a more suitable candidate for cancer cell culture in com- parison to PM-BC-1 and PM-BC-2. Furthermore, PM- BC-3 demonstrated higher tensile properties than PM- BC-1 and comparable to PM-BC-2. Therefore, PM-BC-3 was used in the subsequent cell studies. Pristine BCPM-BC-1PM-BC-2PM-BC-3 0 2 4 6 8 10 12 14 16 Tensile modulus (MPa) (b) # ## 3.4. Cell Viability and Proliferation The MTT assay result shown in Figure 4 demonstrated that cancer cells were viable and the proliferation of MDA-MB-231 cell line was robust, keeping a constant rate during 7 days culture. However, limited viability and proliferation were observed by Szot et al. when cancer cells were cultured on the pristine BC without introduc- tion of macropores [32]. The difference in cell prolifera- tion suggested that the pore structure and size of the tu- mor engineering scaffolds were crucial to the culture of cancer cells. 0 1 2 3 4 5 6 7 8 9 Elongation (%) Pristine BCPM-BC-1PM-BC-2 PM-BC-3 (c) *** 3.5. Morphology of Cells on the PM-BC-3 Scaffold Previous study by Szot et al. demonstrated that cancer cells cultured on BC were not spread out across the sur- face of BC scaffolds due to the absence of manufactured porosity [32]. In this study, adhesion and morphology of the MDA-MB-231 cells after 7 days seeding on the PM- BC-3 scaffold were characterized by SEM and the results are shown in Figure 5. SEM demonstrated that cancer cells were attached to the scaffold. Note that individual cancer cell and cancer cell aggregates attached and spread throughout the surface of the PM-BC-3 scaffold and many cells connected to the neighboring cells as shown in Figures 5(a) and (b). Figures 5(c) and (d) demon- strated a wide spread and tight attachment to the scaffold. Furthermore, the MDA-MB-231 cells on the PM-BC Figure 3. Tensile strength (a), tensile modulus (b), and strain at break (c) of various materials (p+, p#, and p* < 0.05; p## and p** > 0.05). observed because of the creation of macropores, the ten- sile strength value of these PM-BC samples was still much higher than other natural biomaterials. For instance, it was 40-fold higher than hyaluronan-collagen scaffolds (10 - 15 kPa) [49]. Tensile testing indicated that the pre- sence of macropores did not sharply decrease the tensile properties of BC samples, which made the PM-BC scaf-
 A Novel in Vitro Three-Dimensional Macroporous Scaffolds from Bacterial Cellulose for Culture of Breast Cancer Cells 322 01234567 0.0 0.2 0.4 0.6 0.8 1.0 1.2 O.D. (490 nm) Culture time (day) Figure 4. Proliferation of MDA-MB-231 cell line cultured on the PM-BC scaffold by MTT assay. Figure 5. SEM images of MDA-MB-231 cells on the PM-BC scaffold after 2 weeks culture ((a)-(d) showing varying mag- nification). scaffold kept their normal morphology of roughly rounded shape (3D image: oblate spheroid), which was similar to that seen in cells grown on chitosan-alginate (CA) 3D scaffolds reported by Kievit et al. who declared that cells in solid tumors exhibit similar morphology and thus con- cluded that the CA scaffolds could provide a growth en- vironment that promoted the formation of solid tumor- like cells [11]. More importantly, a large number of pro- truded pseudopodiums were formed that bonded to the scaffold (see arrows in Figures 5(c) and (d)), indicating a strong adhesion of cancer cells to the scaffold. It was striking to note that multilayered cancer cells were ob- served on the surface of the PM-BC scaffold (circle in Figure 5(d)) and moreover, cancer cells in the basal layer formed clusters (ellipse in Figure 5(d)), which could never be found for conventional 2D culture due to its limited space. The SEM results suggested that the PM- BC-3 scaffold strongly supported the adhesion, spreading, proliferation, and differentiation of the MDA-MB-231 cell line. 3.6. Histological Observation SEM observation was not able to provide any informa- tion inside the scaffold. In this study, histological obser- vation was performed to evaluate the cells distribution inside the PM-BC scaffold upon culture for varying pe- riods up to 4 weeks. The H&E staining results are shown in Figure 6. Szot and co-workers reported that no cancer cell infiltration was observed after 7 days culture on BC [32] while the results of the present study were totally different. As clearly seen in Figure 6(A), the distribution of the MDA-MB-231 cells within the PM-BC-3 scaffold was observed, suggesting cells migration into the macro- porous scaffold and further formed clusters in some areas (circles in Figures 6(A-b) and (A-c)) even after only 3 days culture. After 7 days culture (Figure 6(B)), the cells spread and grew along the walls of the macropores (see arrows in Figure 6(B)) and more cell clusters were found (see circle in Figure 6(B-c)), indicating successive growth and proliferation inside the PM-BC-3 scaffold. Figures 6(A) to (E) clearly showed an increased cell density and increased number of clusters (Figure 6(E-c) as a repre- sentative) with culture time, indicating that cancer cells experienced robust proliferation, in-growth, and differen- tiation inside the PM-BC-3 scaffold. These findings sug- gested that cancer cells could penetrate into the core of the PM-BC-3 scaffold due to the presence of macropores. The histological observation together with the SEM and MTT results was sufficient to verify that the PM-BC-3 scaffold was able to promote the adhesion, in-growth, proliferation, and differentiation of the MDA-MB-231 cells and thus this macroporous material could be a novel scaffold for the in vitro culture of cancer cells. 4. Conclusion Patterned macroporous BC scaffolds were successfully prepared by a one-step direct perforation in BC hy- drogels by using infrared laser micromachining tech- nique. The PM-BC scaffolds with different pore size and pore density could be obtained. Mechanical tests re- vealed that the PM-BC scaffolds could maintain 88 per- cent tensile strength of the pristine BC scaffolds and in- significant difference in tensile modulus and strain at break were observed between the pristine BC and two PM-BC samples with pore distance of 1.0 mm. All three PM-BC scaffolds prepared in this work showed hierar- chical pore size distribution from several nanometers to over a hundred microns, but the PM-BC-3 scaffold showed the largest pore size (315 µm) and porosity and the wid- est pore size distribution among the three macroporous BC scaffolds. It was concluded that, similar to UV and femtosecond lasers which were suitable for metals and polymers, the infrared laser was an excellent tool for Copyright © 2013 SciRes. JBNB
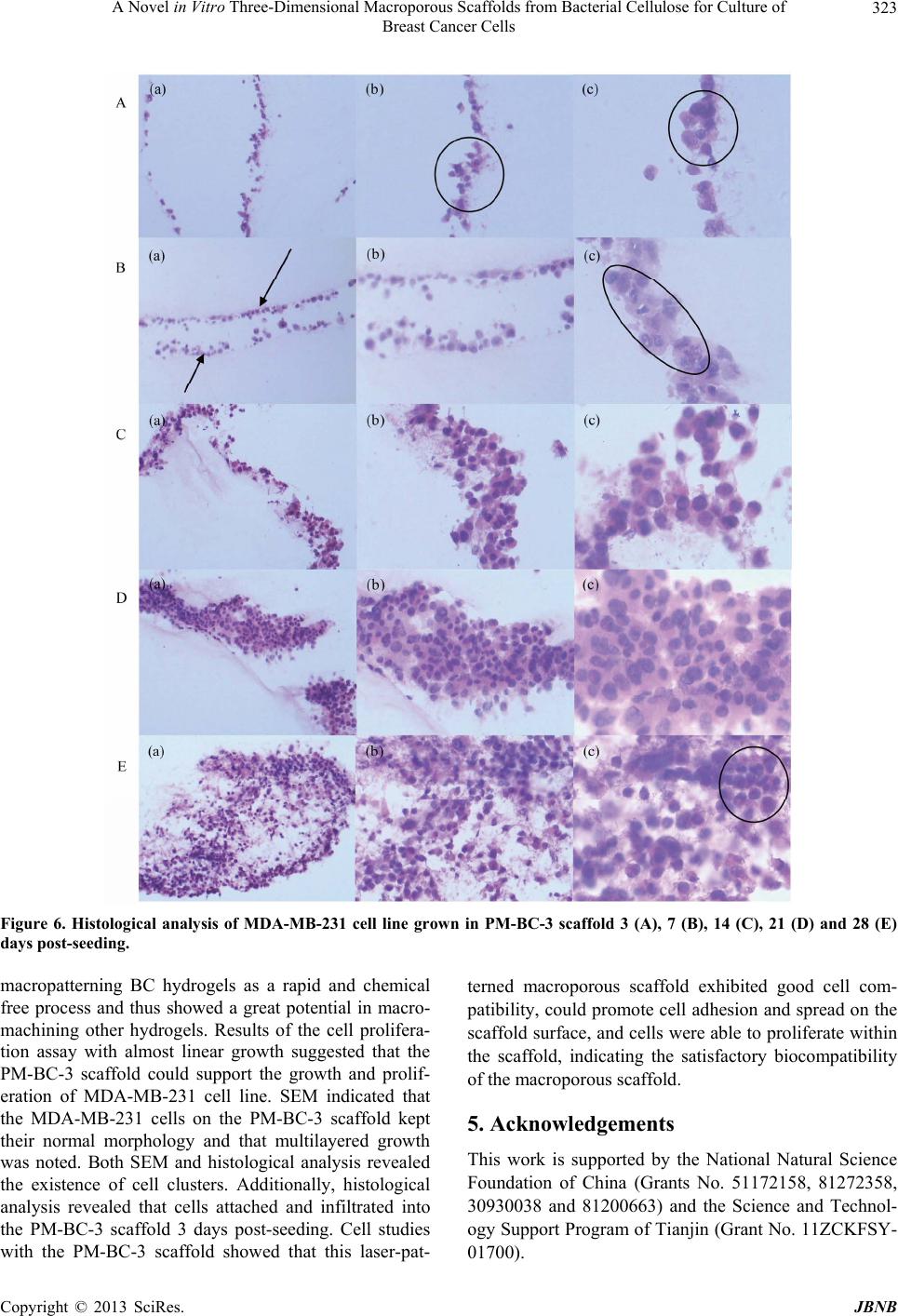 A Novel in Vitro Three-Dimensional Macroporous Scaffolds from Bacterial Cellulose for Culture of Breast Cancer Cells Copyright © 2013 SciRes. JBNB 323 Figure 6. Histological analysis of MDA-MB-231 cell line grown in PM-BC-3 scaffold 3 (A), 7 (B), 14 (C), 21 (D) and 28 (E) days post-seeding. macropatterning BC hydrogels as a rapid and chemical free process and thus showed a great potential in macro- machining other hydrogels. Results of the cell prolifera- tion assay with almost linear growth suggested that the PM-BC-3 scaffold could support the growth and prolif- eration of MDA-MB-231 cell line. SEM indicated that the MDA-MB-231 cells on the PM-BC-3 scaffold kept their normal morphology and that multilayered growth was noted. Both SEM and histological analysis revealed the existence of cell clusters. Additionally, histological analysis revealed that cells attached and infiltrated into the PM-BC-3 scaffold 3 days post-seeding. Cell studies with the PM-BC-3 scaffold showed that this laser-pat- terned macroporous scaffold exhibited good cell com- patibility, could promote cell adhesion and spread on the scaffold surface, and cells were able to proliferate within the scaffold, indicating the satisfactory biocompatibility of the macroporous scaffold. 5. Acknowledgements This work is supported by the National Natural Science Foundation of China (Grants No. 51172158, 81272358, 30930038 and 81200663) and the Science and Technol- ogy Support Program of Tianjin (Grant No. 11ZCKFSY- 01700).
 A Novel in Vitro Three-Dimensional Macroporous Scaffolds from Bacterial Cellulose for Culture of Breast Cancer Cells 324 REFERENCES [1] R. Langer and J. P. Vacanti, “Tissue Engineering,” Sci- ence, Vol. 260, No. 5110, 1993, pp. 920-926. http://dx.doi.org/10.1126/science.8493529 [2] C. M. Ghajar and M. J. Bissell, “Tumor Engineering: The Other Face of Tissue Engineering,” Tissue Engineering Part A, Vol. 16, No. 7, 2010, pp. 2153-2156. http://dx.doi.org/10.1089/ten.tea.2010.0135 [3] Y. Aizawa, S. C. Owen and M. S. Shoichet, “Polymers Used to Influence Cell Fate in 3D Geometry: New Trends,” Progress in Polymer Science, Vol. 37, No. 5, 2012, pp. 645-658. http://dx.doi.org/10.1016/j.progpolymsci.2011.11.004 [4] G. Y. Lee, P. A. Kenny, E. H. Lee and M. J. Bissell, “Three-Dimensional Culture Models of Normal and Ma- lignant Breast Epithelial Cells,” Nature Methods, Vol. 4, No. 4, 2007, pp. 359-365. http://dx.doi.org/10.1038/nmeth1015 [5] C. Fischbach, R. Chen, T. Matsumoto, T. Schmelzle, J. S. Brugge, P. J. Polverini and D. J. Mooney, “Engineering Tumors with 3D Scaffolds,” Nature Methods, Vol. 4, No. 10, 2007, pp. 855-860. http://dx.doi.org/10.1038/nmeth1085 [6] M. A. Cichon, V. G. Gainullin, Y. Zhang and D. C. Ra- disky, “Growth of Lung Cancer Cells in Three-Dimen- sional Microenvironments Reveals Key Features of Tu- mor Malignancy,” Integrative Biology, Vol. 4, No. 4, 2012, pp. 440-448. http://dx.doi.org/10.1039/c1ib00090j [7] J. L. Horning, S. K. Sahoo, S. Vijayaraghavalu, S. Dimi- trijevic, J. K. Vasir, T. K. Jain, A. K. Panda and V. Lab- hasetwar, “3-D Tumor Model for in Vitro Evaluation of Anticancer Drugs,” Molecular Pharmacology, Vol. 5, No. 5, 2008, pp. 849-862. http://dx.doi.org/10.1021/mp800047v [8] M. Mitra, C. Mohanty, A. Harilal, U. K. Maheswari, S. K. Sahoo and S. Krishnakumar, “A Novel in Vitro Three- Dimensional Retinoblastoma Model for Evaluating Che- motherapeutic Drugs,” Molecular Vision, Vol. 18, No. 142-145, 2012, pp. 1361-1378. [9] L. Chen, Z. Xiao, Y. Meng, Y. Zhao, J. Han, G. Su, B. Chen and J. Dai, “The Enhancement of Cancer Stem Cell Properties of MCF-7 Cells in 3D Collagen Scaffolds for Modeling of Cancer and Anti-Cancer Drugs,” Biomate- rials, Vol. 33, No. 5, 2012, pp. 1437-1444. http://dx.doi.org/10.1016/j.biomaterials.2011.10.056 [10] C. S. Szot, C. F. Buchanan, J. W. Freeman and M. N. Rylander, “3D in Vitro Bioengineered Tumors Based on Collagen I Hydrogels,” Biomaterials, Vol. 32, No. 31, 2011, pp. 7905-7912. http://dx.doi.org/10.1016/j.biomaterials.2011.07.001 [11] F. M. Kievit, S. J. Florczyk, M. C. Leung, O. Veiseh, J. O. Park, M. L. Disis and M. Zhang, “Chitosan-Alginate 3D Scaffolds as a Mimic of the Glioma Tumor Microenvi- ronment,” Biomaterials, Vol. 31, No. 22, 2010, pp. 5903- 5910. http://dx.doi.org/10.1016/j.biomaterials.2010.03.062 [12] K. A. Beningo, M. Dembo and Y. Wang, “Responses of Fibroblasts to Anchorage of Dorsal Extracellular Matrix Receptors,” Proceedings of the National Academy of Sci- ences of the United States of America, Vol. 101, No. 52, 2004, p. 18024. http://dx.doi.org/10.1073/pnas.0405747102 [13] S. K. Sahoo, A. K. Panda and V. Labhasetwar, “Charac- terization of Porous PLGA/PLA Microparticles as a Scaf- fold for Three Dimensional Growth of Breast Cancer Cells,” Biomacromolecules, Vol. 6, No. 2, 2005, pp. 1132-1139. http://dx.doi.org/10.1021/bm0492632 [14] N. Rhodes, J. Srivastava, R. Smith and C. Longinotti, “Metabolic and Histological Analysis of Mesenchymal Stem Cells Grown in 3-D Hyaluronan-Based Scaffolds,” Journal of Materials Science: Materials in Medicine, Vol. 15, No. 4, 2004, pp. 391-395. http://dx.doi.org/10.1023/B:JMSM.0000021108.74004.7e [15] S. Talukdar, M. Mandal, D. W. Hutmacher, P. J. Russell, C. Soekmadji and S. C. Kundu, “Engineered Silk Fibroin Protein 3D Matrices for in Vitro Tumor Model,” Bioma- terials, Vol. 32, No. 8, 2010, pp. 2149-2159. http://dx.doi.org/10.1016/j.biomaterials.2010.11.052 [16] J. Lannutti, D. Reneker, T. Ma, D. Tomasko and D. F. Farson, “Electrospinning for Tissue Engineering Scaf- folds,” Materials Science and Engineering C, Vol. 27, No. 3, 2007, pp. 504-509. http://dx.doi.org/10.1016/j.msec.2006.05.019 [17] S. Agarwal, J. H. Wendorff and A. Greiner, “Progress in the Field of Electrospinning for Tissue Engineering Ap- plications,” Advanced Materials, Vol. 21, No. 32-33, 2009, pp. 3343-3351. http://dx.doi.org/10.1002/adma.200803092 [18] W. Yang, F. Yang, Y. Wang, S. K. Both and J. A. Jansen, “In Vivo Bone Generation via the Endochondral Pathway on Three-Dimensional Electrospun Fibers,” Acta Bioma- terialia, Vol. 9, No. 1, 2013, pp. 4505-4512. http://dx.doi.org/10.1016/j.actbio.2012.10.003 [19] T. Dvir, B. P. Timko, D. S. Kohane and R. Langer, “Nanotechnological Strategies for Engineering Complex Tissues,” Nature Nanotechnology, Vol. 6, No. 1, 2011, pp. 13-22. http://dx.doi.org/10.1038/nnano.2010.246 [20] T. G. Kim, H. Shin and D. W. Lim, “Biomimetic Scaf- folds for Tissue Engineering,” Advanced Functional Ma- terials, Vol. 22, No. 12, 2012, pp. 2446-2468. http://dx.doi.org/10.1002/adfm.201103083 [21] C. Vaquette and J. Cooper-White, “A Simple Method for Fabricating 3-D Multilayered Composite Scaffolds,” Acta Biomaterialia, Vol. 9, No. 1, 2013, pp. 4599-4608. http://dx.doi.org/10.1016/j.actbio.2012.08.015 [22] J. Mao, S. Duan, A. Song, Q. Cai, X. Deng and X. Yang, “Macroporous and Nanofibrous Poly(Lactide-co-Glyco- lide)(50/50) Scaffolds via Phase Separation Combined with Particle-Leaching,” Materials Science and Engineer- ing C, Vol. 32, No. 6, 2012, pp. 1407-1414. http://dx.doi.org/10.1016/j.msec.2012.04.018 [23] N. Petersen and P. Gatenholm, “Bacterial Cellulose- Based Materials and Medical Devices: Current State and Perspectives,” Applied Microbiology and Biotechnology, Vol. 91, No. 5, 2011, pp. 1277-1286. http://dx.doi.org/10.1007/s00253-011-3432-y Copyright © 2013 SciRes. JBNB
 A Novel in Vitro Three-Dimensional Macroporous Scaffolds from Bacterial Cellulose for Culture of Breast Cancer Cells 325 [24] D. Klemm, F. Kramer, S. Moritz, T. Lindstrom, M. An- kerfors, D. Gray and A. Dorris, “Nanocelluloses: A New Family of Nature-Based Materials,” Angewandte Chemie International Edition, Vol. 50, No. 24, 2011, pp. 5438- 5466. http://dx.doi.org/10.1002/anie.201001273 [25] S. Tanpichai, F. Quero, M. Nogi, H. Yano, R. J. Young, T. Lindstrom, W. W. Sampson and S. J. Eichhorn, “Effec- tive Young’s Modulus of Bacterial and Microfibrillated Cellulose Fibrils in Fibrous Networks,” Biomacromole- cules, Vol. 13, No. 5, 2012, pp. 1340-1349. http://dx.doi.org/10.1021/bm300042t [26] R. A. N. Pertile, S. Moreira, R. M. Gil da Costa, A. Cor- reia, L. Guardao, F. Gartner, M. Vilanova and M. Gama, “Bacterial Cellulose: Long-Term Biocompatibility Stud- ies,” Journal of Biomaterials Science Polymer Edition, Vol. 23, No. 10, 2012, pp. 1339-1354. [27] F. K. Andrade, N. Alexandre, I. Amorim, F. Gartner, A. C. Maurício, A. L. Luís and M. Gama1, “Studies on the Biocompatibility of Bacterial Cellulose,” Journal of Bio- active and Compatable Polymers, Vol. 28, No. 1, 2013, pp. 97-112. [28] P. M. Favi, R. S. Benson, N. R. Neilsen, R. L. Hammonds, C. C. Bates, C. P. Stephens and M. S. Dhar, “Cell Prolif- eration, Viability, and in Vitro Differentiation of Equine Mesenchymal Stem Cells Seeded on Bacterial Cellulose Hydrogel Scaffolds,” Materials Science and Engineering C, Vol. 33, No. 4, 2013, pp. 1935-1944. http://dx.doi.org/10.1016/j.msec.2012.12.100 [29] Q. Shi, Y. Li, J. Sun, H. Zhang, L. Chen, B. Chen, H. Yang and Z. Wang, “The Osteogenesis of Bacterial Cel- lulose Scaffold Loaded with Bone Morphogenetic Pro- tein-2,” Biomaterials, Vol. 33, No. 28, 2012, pp. 6644- 6649. http://dx.doi.org/10.1016/j.biomaterials.2012.05.071 [30] S. Saska, R. M. Scarel-Caminaga, L. N. Teixeira, L. P. Franchi, R. A. Dos Santos, A. M. M. Gaspar, P. T. de Oliveira, A. L. Rosa, C. S. Takahashi, Y. Messaddeq, S. J. L. Ribeiro and R. Marchetto, “Characterization and in Vi- tro Evaluation of Bacterial Cellulose Membranes Func- tionalized with Osteogenic Growth Peptide for Bone Tis- sue Engineering,” Journal of Materials Science Materials in Medici ne, Vol. 23, No. 9, 2012, pp. 2253-2266. http://dx.doi.org/10.1007/s10856-012-4676-5 [31] K. Hirayama, T. Okitsu, H. Teramae, D. Kiriya, H. Onoe and S. Takeuchi, “Cellular Building Unit Integrated with Microstrand-Shaped Bacterial Cellulose,” Biomaterials, Vol. 34, No. 10, 2013, pp. 2421-2427. http://dx.doi.org/10.1016/j.biomaterials.2012.12.013 [32] C. S. Szot, C. F. Buchanan, P. Gatenholm, M. N. Ry- lander and J. W. Freeman, “Investigation of Cancer Cell Behavior on Nanofibrous Scaffolds,” Materials Science and Engineering C, Vol. 31, No. 1, 2011, pp. 37-42. http://dx.doi.org/10.1016/j.msec.2009.12.005 [33] J. M. Taboas, R. D. Maddox, P. H. Krebsbach and S. J. Hollister, “Indirect Solid Free form Fabrication of Local and Global Porous, Biomimetic and Composite 3D Poly- mer-Ceramic Scaffolds,” Biomaterials, Vol. 24, No. 1, 2003, pp. 181-194. http://dx.doi.org/10.1016/S0142-9612(02)00276-4 [34] C. Martinez-Ramos, A. Valles-Lluch, J. M. Garcia Ver- dugo, J. L. Gomez Ribelles, J. Antonio Barcia, A. Baiget Orts, J. M. Soria Lopez and M. Monleon Pradas, “Chan- neled Scaffolds Implanted in Adult Rat Brain,” Journal of Biomedial Materials Research Part A, Vol. 100A, No. 12, 2012, pp. 3276-3286. [35] J. Wang, C. Yang, Y. Wan, H. Luo, F. He, K. Dai and Y. Huang, “Laser Patterning of Bacterial Cellulose Hydrogel and Its Modification with Gelatin and Hydroxyapatite for Bone Tissue Engineering,” Soft Materials, Vol. 11, No. 2, 2013, pp. 173-180. http://dx.doi.org/10.1080/1539445X.2011.611204 [36] J. C. Le Huec, T. Schaeverbeke, D. Clement, J. Faber and A. Le Rebeller, “Influence of Porosity on the Mechanical Resistance of Hydroxyapatite Ceramics under Compres- sive Stress,” Biomaterials, Vol. 16, No. 2, 1995, pp. 113- 118. http://dx.doi.org/10.1016/0142-9612(95)98272-G [37] L. Hong, Y. L. Wang, S. R. Jia, Y. Huang, C. Gao and Y. Z. Wan, “Hydroxyapatite/Bacterial Cellulose Composites Synthesized via a Biomimetic Route,” Materials Letters, Vol. 60, No. 13-14, 2006, pp. 1710-1713. http://dx.doi.org/10.1016/j.matlet.2005.12.004 [38] Y. Z. Wan, L. Hong, S. R. Jia, Y. Huang, Y. Zhu, Y. L. Wang and H. J. Jiang, “Synthesis and Characterization of Hydroxyapatite-Bacterial Cellulose Nanocomposites,” Com- posites Science and Technology, Vol. 66, No. 11-12, 2006, pp. 1825-1832. http://dx.doi.org/10.1016/j.compscitech.2005.11.027 [39] A. I. Itala, H. O. Ylanen, C. Ekholm, K. H. Karlsson and H. T. Aro, “Pore Diameter of More than 100 mu m Is Not Requisite for Bone Ingrowth in Rabbits,” Journal of Bio- medial Materials Research, Vol. 58, No. 6, 2001, pp. 679-683. http://dx.doi.org/10.1002/jbm.1069 [40] H. E. Gotz, M. Muller, A. Emmel, U. Holzwarth, R. G. Erben and R. Stangl, “Effect of Surface Finish on the Os- seointegration of Laser-Treated Titanium Alloy Implants,” Biomaterials, Vol. 25, No. 18, 2004, pp. 4057-4064. http://dx.doi.org/10.1016/j.biomaterials.2003.11.002 [41] V. V. Kancharla and S. C. Chen, “Fabrication of Biode- gradable Polymeric Micro-Devices Using Laser Micro- machining,” Biomedical Mic rodevices, Vol. 4, No. 2, 2002, pp. 105-109. http://dx.doi.org/10.1023/A:1014679013888 [42] S. Chen, V. V. Kancharla and Y. Lu, “Laser-Based Mi- croscale Patterning of Biodegradable Polymers for Bio- medical Applications,” International Journal of Materials and Product Technology, Vol. 18, No. 4-6, 2003, pp. 457- 468. [43] C. A. Aguilar, Y. Lu, S. Mao and S. C. Chen, “Direct Micro-Patterning of Biodegradable Polymers Using Ul- traviolet and Femtosecond Lasers,” Biomaterials, Vol. 26, No. 36, 2005, pp. 7642-7649. http://dx.doi.org/10.1016/j.biomaterials.2005.04.053 [44] C. Gao, Y. Z. Wan, C. X. Yang, K. R. Dai, T. T. Tang, H. L. Luo and J. H. Wang, “Preparation and Characterization of Bacterial Cellulose Sponge with Hierarchical Pore Structure as Tissue Engineering Scaffold,” Journal of Porous Materials, Vol. 18, 2011, pp. 139-145. http://dx.doi.org/10.1007/s10934-010-9364-6 Copyright © 2013 SciRes. JBNB
 A Novel in Vitro Three-Dimensional Macroporous Scaffolds from Bacterial Cellulose for Culture of Breast Cancer Cells Copyright © 2013 SciRes. JBNB 326 [45] V. Karageorgiou and D. Kaplan, “Porosity of 3D Bior- naterial Scaffolds and Osteogenesis,” Biomaterials, Vol. 26, No. 27, 2005, pp. 5474-5491. http://dx.doi.org/10.1016/j.biomaterials.2005.02.002 [46] M. Flaibani and N. Elvassore, “Gas Anti-Solvent Precipi- tation Assisted Salt Leaching for Generation of Micro- and Nano-Porous Wall in Bio-Polymeric 3D Scaffolds,” Materials Science and Engineering C, Vol. 32, No. 6, 2012, pp. 1632-1639. http://dx.doi.org/10.1016/j.msec.2012.04.054 [47] N. D. Evans, E. Gentleman and J. M. Polak, “Scaffolds for Stem Cells,” Materials Today, Vol. 9, No. 12, 2006, pp. 26-33. http://dx.doi.org/10.1016/S1369-7021(06)71740-0 [48] B. J. Papenburg, J. Liu, G. A. Higuera, A. M. C. Barradas, J. de Boer, C. A. van Blitterswijk, M. Wessling and D. Stamatialis, “Development and Analysis of Multi-Layer Scaffolds for Tissue Engineering,” Biomaterials, Vol. 30, No. 31, 2009, pp. 6228-6239. http://dx.doi.org/10.1016/j.biomaterials.2009.07.057 [49] A. A. Al-Munajjed, M. Hien, R. Kujat, J. P. Gleeson and J. Hammer, “Influence of Pore Size on Tensile Strength, Permeability and Porosity of Hyaluronan-Collagen Scaf- folds,” Journal of Materials Science Materials in Medi- cine, Vol. 19, No. 8, 2008, pp. 2859-2864. http://dx.doi.org/10.1007/s10856-008-3422-5 [50] E. Tsuruga, H. Takita, H. Itoh, Y. Wakisaka and Y. Ku- boki, “Pore Size of Porous Hydroxyapatite as the Cell- Substratum Controls BMP-Induced Osteogenesis,” Jour- nal of Biochemistry, Vol. 121, No. 2, 1997, pp. 317-324. http://dx.doi.org/10.1093/oxfordjournals.jbchem.a021589
|