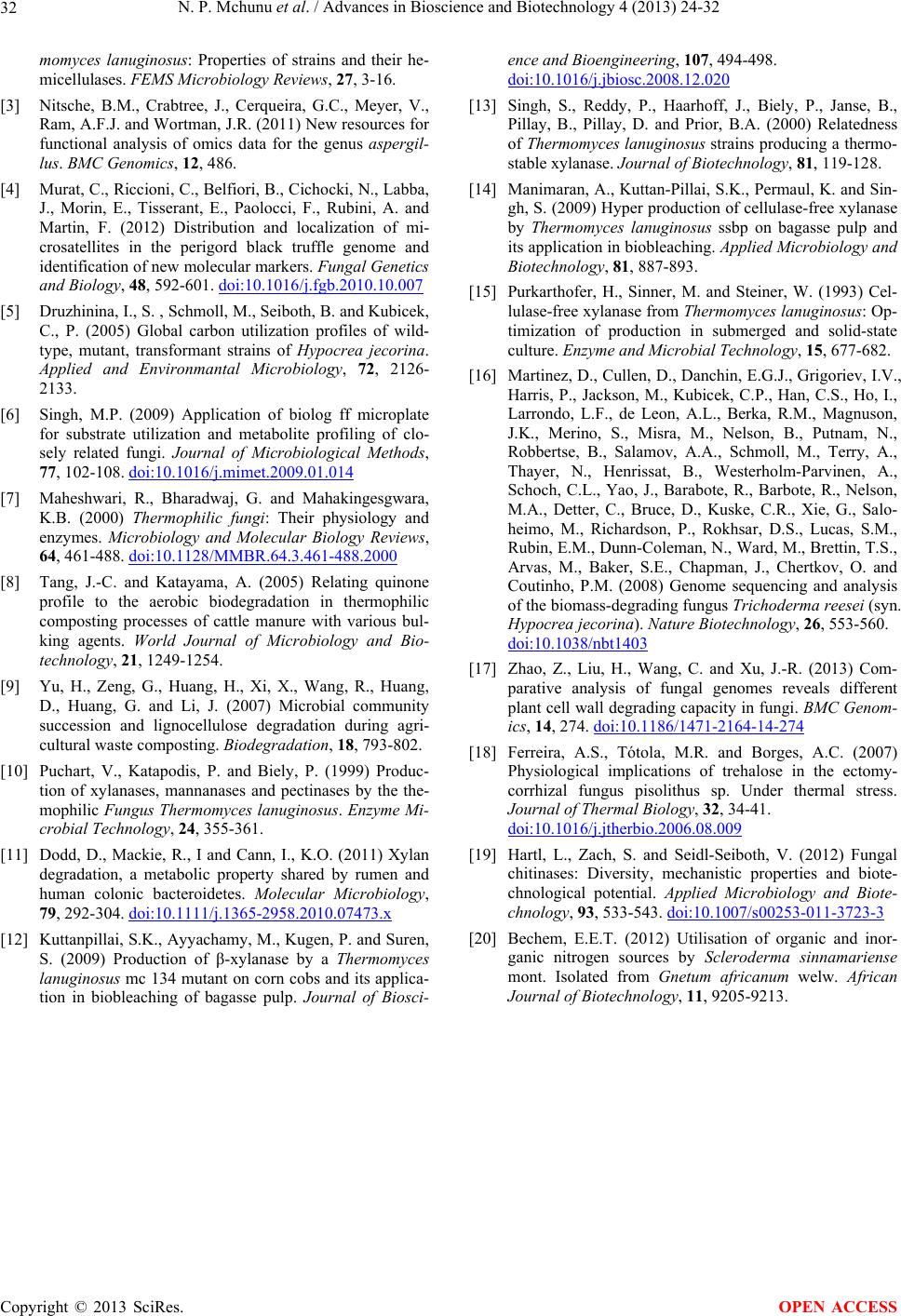
N. P. Mchunu et al. / Advances in Bioscience and Biotechnology 4 (2013) 24-32
32
momyces lanuginosus: Properties of strains and their he-
micellulases. FEMS Microbiology Reviews, 27, 3-16.
[3] Nitsche, B.M., Crabtree, J., Cerqueira, G.C., Meyer, V.,
Ram, A.F.J. and Wortman, J.R. (2011) New resources for
functional analysis of omics data for the genus aspergil-
lus. BMC Genomics, 12, 486.
[4] Murat, C., Riccioni, C., Belfiori, B., Cichocki, N., Labba,
J., Morin, E., Tisserant, E., Paolocci, F., Rubini, A. and
Martin, F. (2012) Distribution and localization of mi-
crosatellites in the perigord black truffle genome and
identification of new molecular markers. Fungal Genetics
and Biology, 48, 592-601. doi:10.1016/j.fgb.2010.10.007
[5] Druzhinina, I., S. , Schmoll, M., Seiboth, B. and Kubicek,
C., P. (2005) Global carbon utilization profiles of wild-
type, mutant, transformant strains of Hypocrea jecorina.
Applied and Environmantal Microbiology, 72, 2126-
2133.
[6] Singh, M.P. (2009) Application of biolog ff microplate
for substrate utilization and metabolite profiling of clo-
sely related fungi. Journal of Microbiological Methods,
77, 102-108. doi:10.1016/j.mimet.2009.01.014
[7] Maheshwari, R., Bharadwaj, G. and Mahakingesgwara,
K.B. (2000) Thermophilic fungi: Their physiology and
enzymes. Microbiology and Molecular Biology Reviews,
64, 461-488. doi:10.1128/MMBR.64.3.461-488.2000
[8] Tang, J.-C. and Katayama, A. (2005) Relating quinone
profile to the aerobic biodegradation in thermophilic
composting processes of cattle manure with various bul-
king agents. World Journal of Microbiology and Bio-
technology, 21, 1249-1254.
[9] Yu, H., Zeng, G., Huang, H., Xi, X., Wang, R., Huang,
D., Huang, G. and Li, J. (2007) Microbial community
succession and lignocellulose degradation during agri-
cultural waste composting. Biodegradation, 18, 793-802.
[10] Puchart, V., Katapodis, P. and Biely, P. (1999) Produc-
tion of xylanases, mannanases and pectinases by the the-
mophilic Fungus Thermomyces lanuginosus. Enzyme Mi-
crobial Technology, 24, 355-361.
[11] Dodd, D., Mackie, R., I and Cann, I., K.O. (2011) Xylan
degradation, a metabolic property shared by rumen and
human colonic bacteroidetes. Molecular Microbiology,
79, 292-304. doi:10.1111/j.1365-2958.2010.07473.x
[12] Kuttanpillai, S.K., Ayyachamy, M., Kugen, P. and Suren,
S. (2009) Production of β-xylanase by a Thermomyces
lanuginosus mc 134 mutant on corn cobs and its applica-
tion in biobleaching of bagasse pulp. Journal of Biosci-
ence and Bioengineering, 107, 494-498.
doi:10.1016/j.jbiosc.2008.12.020
[13] Singh, S., Reddy, P., Haarhoff, J., Biely, P., Janse, B.,
Pillay, B., Pillay, D. and Prior, B.A. (2000) Relatedness
of Thermomyces lanuginosus strains producing a thermo-
stable xylanase. Journal of Biotechnology, 81, 119-128.
[14] Manimaran, A., Kuttan-Pillai, S.K., Permaul, K. and Sin-
gh, S. (2009) Hyper production of cellulase-free xylanase
by Thermomyces lanuginosus ssbp on bagasse pulp and
its application in biobleaching. Applied Microbiology and
Biotechnology, 81, 887-893.
[15] Purkarthofer, H., Sinner, M. and Steiner, W. (1993) Cel-
lulase-free xylanase from Thermomyces lanuginosus: Op-
timization of production in submerged and solid-state
culture. Enzyme and Microbial Technology, 15, 677-682.
[16] Martinez, D., Cullen, D., Danchin, E.G.J., Grigoriev, I.V.,
Harris, P., Jackson, M., Kubicek, C.P., Han, C.S., Ho, I.,
Larrondo, L.F., de Leon, A.L., Berka, R.M., Magnuson,
J.K., Merino, S., Misra, M., Nelson, B., Putnam, N.,
Robbertse, B., Salamov, A.A., Schmoll, M., Terry, A.,
Thayer, N., Henrissat, B., Westerholm-Parvinen, A.,
Schoch, C.L., Yao, J., Barabote, R., Barbote, R., Nelson,
M.A., Detter, C., Bruce, D., Kuske, C.R., Xie, G., Salo-
heimo, M., Richardson, P., Rokhsar, D.S., Lucas, S.M.,
Rubin, E.M., Dunn-Coleman, N., Ward, M., Brettin, T.S.,
Arvas, M., Baker, S.E., Chapman, J., Chertkov, O. and
Coutinho, P.M. (2008) Genome sequencing and analysis
of the biomass-degrading fungus Trichoderma reesei (syn.
Hypocrea jecorina). Nature Biotechnology, 26, 553-560.
doi:10.1038/nbt1403
[17] Zhao, Z., Liu, H., Wang, C. and Xu, J.-R. (2013) Com-
parative analysis of fungal genomes reveals different
plant cell wall degrading capacity in fungi. BMC Genom-
ics, 14, 274. doi:10.1186/1471-2164-14-274
[18] Ferreira, A.S., Tótola, M.R. and Borges, A.C. (2007)
Physiological implications of trehalose in the ectomy-
corrhizal fungus pisolithus sp. Under thermal stress.
Journal of Thermal Biology, 32, 34-41.
doi:10.1016/j.jtherbio.2006.08.009
[19] Hartl, L., Zach, S. and Seidl-Seiboth, V. (2012) Fungal
chitinases: Diversity, mechanistic properties and biote-
chnological potential. Applied Microbiology and Biote-
chnology, 93, 533-543. doi:10.1007/s00253-011-3723-3
[20] Bechem, E.E.T. (2012) Utilisation of organic and inor-
ganic nitrogen sources by Scleroderma sinnamariense
mont. Isolated from Gnetum africanum welw. African
Journal of Biotechnology, 11, 9205-9213.
Copyright © 2013 SciRes. OPEN ACCESS