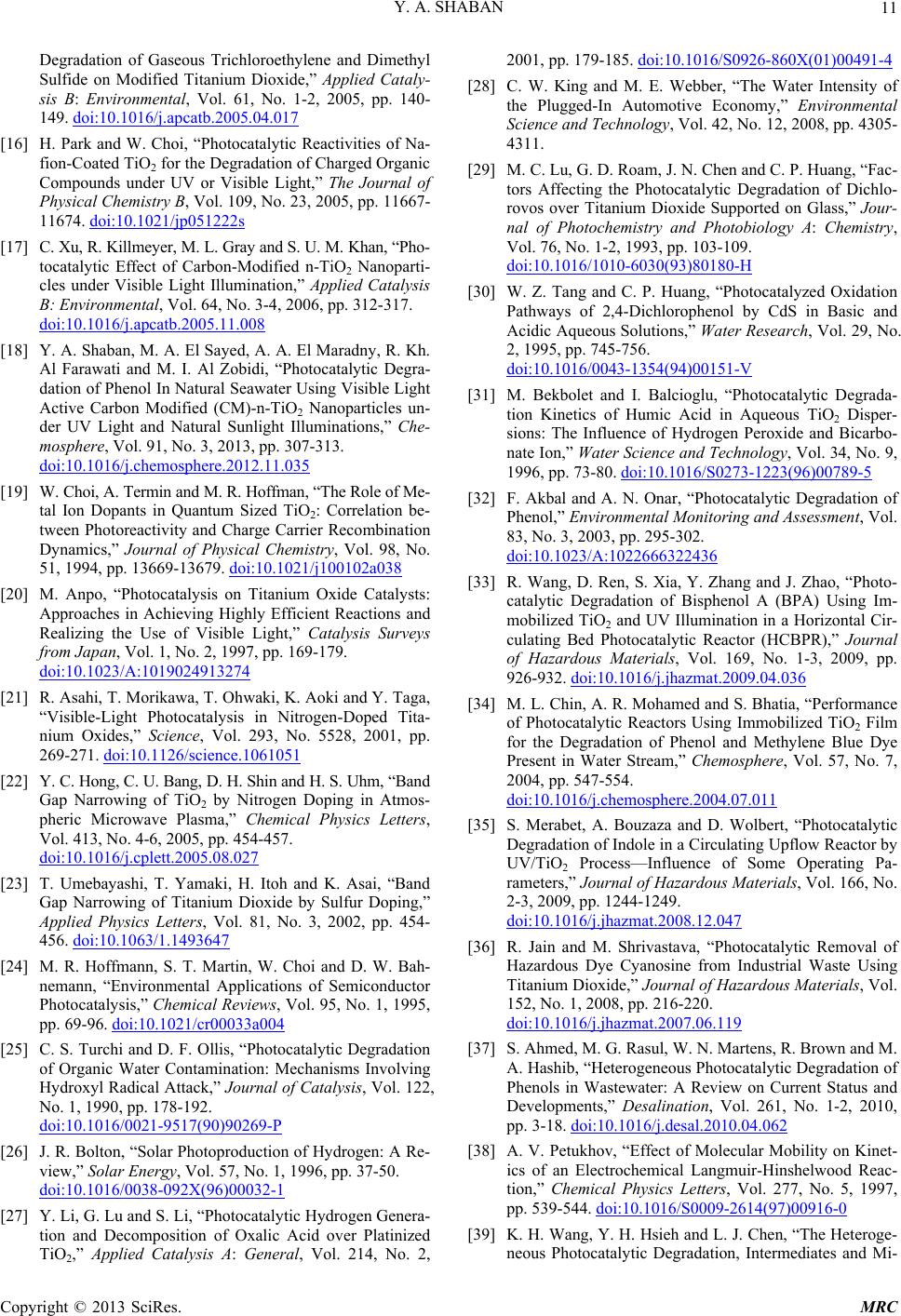
Y. A. SHABAN 11
Degradation of Gaseous Trichloroethylene and Dimethyl
Sulfide on Modified Titanium Dioxide,” Applied Cataly-
sis B: Environmental, Vol. 61, No. 1-2, 2005, pp. 140-
149. doi:10.1016/j.apcatb.2005.04.017
[16] H. Park and W. Choi, “Photocatalytic Reactivities of Na-
fion-Coated TiO2 for the Degradation of Charged Organic
Compounds under UV or Visible Light,” The Journal of
Physical Chemistry B, Vol. 109, No. 23, 2005, pp. 11667-
11674. doi:10.1021/jp051222s
[17] C. Xu, R. Killmeyer, M. L. Gray and S. U. M. Khan, “Pho-
tocatalytic Effect of Carbon-Modified n-TiO2 Nanoparti-
cles under Visible Light Illumination,” Applied Catalysis
B: Environmental, Vol. 64, No. 3-4, 2006, pp. 312-317.
doi:10.1016/j.apcatb.2005.11.008
[18] Y. A. Shaban, M. A. El Sayed, A. A. El Maradny, R. Kh.
Al Farawati and M. I. Al Zobidi, “Photocatalytic Degra-
dation of Phenol In Natural Seawater Using Visible Light
Active Carbon Modified (CM)-n-TiO2 Nanoparticles un-
der UV Light and Natural Sunlight Illuminations,” Che-
mosphere, Vol. 91, No. 3, 2013, pp. 307-313.
doi:10.1016/j.chemosphere.2012.11.035
[19] W. Choi, A. Termin and M. R. Hoffman, “The Role of Me-
tal Ion Dopants in Quantum Sized TiO2: Correlation be-
tween Photoreactivity and Charge Carrier Recombination
Dynamics,” Journal of Physical Chemistry, Vol. 98, No.
51, 1994, pp. 13669-13679. doi:10.1021/j100102a038
[20] M. Anpo, “Photocatalysis on Titanium Oxide Catalysts:
Approaches in Achieving Highly Efficient Reactions and
Realizing the Use of Visible Light,” Catalysis Surveys
from Japan, Vol. 1, No. 2, 1997, pp. 169-179.
doi:10.1023/A:1019024913274
[21] R. Asahi, T. Morikawa, T. Ohwaki, K. Aoki and Y. Taga,
“Visible-Light Photocatalysis in Nitrogen-Doped Tita-
nium Oxides,” Science, Vol. 293, No. 5528, 2001, pp.
269-271. doi:10.1126/science.1061051
[22] Y. C. Hong, C. U. Bang, D. H. Shin and H. S. Uhm, “Band
Gap Narrowing of TiO2 by Nitrogen Doping in Atmos-
pheric Microwave Plasma,” Chemical Physics Letters,
Vol. 413, No. 4-6, 2005, pp. 454-457.
doi:10.1016/j.cplett.2005.08.027
[23] T. Umebayashi, T. Yamaki, H. Itoh and K. Asai, “Band
Gap Narrowing of Titanium Dioxide by Sulfur Doping,”
Applied Physics Letters, Vol. 81, No. 3, 2002, pp. 454-
456. doi:10.1063/1.1493647
[24] M. R. Hoffmann, S. T. Martin, W. Choi and D. W. Bah-
nemann, “Environmental Applications of Semiconductor
Photocatalysis,” Chemical Reviews, Vol. 95, No. 1, 1995,
pp. 69-96. doi:10.1021/cr00033a004
[25] C. S. Turchi and D. F. Ollis, “Photocatalytic Degradation
of Organic Water Contamination: Mechanisms Involving
Hydroxyl Radical Attack,” Journal of Catalysis, Vol. 122,
No. 1, 1990, pp. 178-192.
doi:10.1016/0021-9517(90)90269-P
[26] J. R. Bolton, “Solar Photoproduction of Hydrogen: A Re-
view,” Solar Energy, Vol. 57, No. 1, 1996, pp. 37-50.
doi:10.1016/0038-092X(96)00032-1
[27] Y. Li, G. Lu and S. Li, “Photocatalytic Hydrogen Genera-
tion and Decomposition of Oxalic Acid over Platinized
TiO2,” Applied Catalysis A: General, Vol. 214, No. 2,
2001, pp. 179-185. doi:10.1016/S0926-860X(01)00491-4
[28] C. W. King and M. E. Webber, “The Water Intensity of
the Plugged-In Automotive Economy,” Environmental
Science and Technology, Vol. 42, No. 12, 2008, pp. 4305-
4311.
[29] M. C. Lu, G. D. Roam, J. N. Chen and C. P. Huang, “Fac-
tors Affecting the Photocatalytic Degradation of Dichlo-
rovos over Titanium Dioxide Supported on Glass,” Jour-
nal of Photochemistry and Photobiology A: Chemistry,
Vol. 76, No. 1-2, 1993, pp. 103-109.
doi:10.1016/1010-6030(93)80180-H
[30] W. Z. Tang and C. P. Huang, “Photocatalyzed Oxidation
Pathways of 2,4-Dichlorophenol by CdS in Basic and
Acidic Aqueous Solutions,” Water Research, Vol. 29, No.
2, 1995, pp. 745-756.
doi:10.1016/0043-1354(94)00151-V
[31] M. Bekbolet and I. Balcioglu, “Photocatalytic Degrada-
tion Kinetics of Humic Acid in Aqueous TiO2 Disper-
sions: The Influence of Hydrogen Peroxide and Bicarbo-
nate Ion,” Water Science and Technology, Vol. 34, No. 9,
1996, pp. 73-80. doi:10.1016/S0273-1223(96)00789-5
[32] F. Akbal and A. N. Onar, “Photocatalytic Degradation of
Phenol,” Environmental Monitoring and Assessment, Vol.
83, No. 3, 2003, pp. 295-302.
doi:10.1023/A:1022666322436
[33] R. Wang, D. Ren, S. Xia, Y. Zhang and J. Zhao, “Photo-
catalytic Degradation of Bisphenol A (BPA) Using Im-
mobilized TiO2 and UV Illumination in a Horizontal Cir-
culating Bed Photocatalytic Reactor (HCBPR),” Journal
of Hazardous Materials, Vol. 169, No. 1-3, 2009, pp.
926-932. doi:10.1016/j.jhazmat.2009.04.036
[34] M. L. Chin, A. R. Mohamed and S. Bhatia, “Performance
of Photocatalytic Reactors Using Immobilized TiO2 Film
for the Degradation of Phenol and Methylene Blue Dye
Present in Water Stream,” Chemosphere, Vol. 57, No. 7,
2004, pp. 547-554.
doi:10.1016/j.chemosphere.2004.07.011
[35] S. Merabet, A. Bouzaza and D. Wolbert, “Photocatalytic
Degradation of Indole in a Circulating Upflow Reactor by
UV/TiO2 Process—Influence of Some Operating Pa-
rameters,” Journal of Hazardous Materials, Vol. 166, No.
2-3, 2009, pp. 1244-1249.
doi:10.1016/j.jhazmat.2008.12.047
[36] R. Jain and M. Shrivastava, “Photocatalytic Removal of
Hazardous Dye Cyanosine from Industrial Waste Using
Titanium Dioxide,” Journal of Hazardous Materials, Vol.
152, No. 1, 2008, pp. 216-220.
doi:10.1016/j.jhazmat.2007.06.119
[37] S. Ahmed, M. G. Rasul, W. N. Martens, R. Brown and M.
A. Hashib, “Heterogeneous Photocatalytic Degradation of
Phenols in Wastewater: A Review on Current Status and
Developments,” Desalination, Vol. 261, No. 1-2, 2010,
pp. 3-18. doi:10.1016/j.desal.2010.04.062
[38] A. V. Petukhov, “Effect of Molecular Mobility on Kinet-
ics of an Electrochemical Langmuir-Hinshelwood Reac-
tion,” Chemical Physics Letters, Vol. 277, No. 5, 1997,
pp. 539-544. doi:10.1016/S0009-2614(97)00916-0
[39] K. H. Wang, Y. H. Hsieh and L. J. Chen, “The Heteroge-
neous Photocatalytic Degradation, Intermediates and Mi-
Copyright © 2013 SciRes. MRC