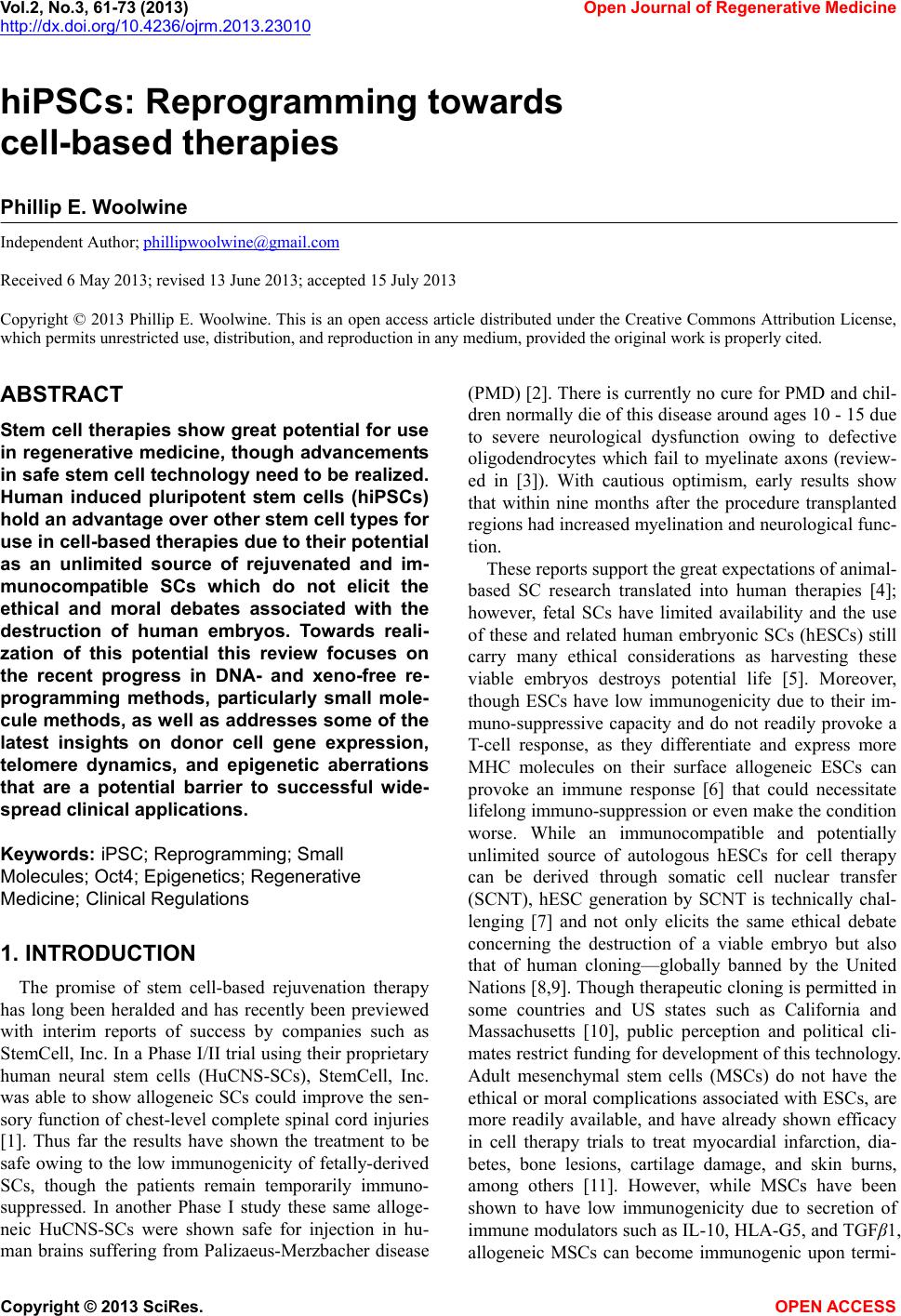 Vol.2, No.3, 61-73 (2013) Open Journal of Regenerativ e Medicine http://dx.doi.org/10.4236/ojrm.2013.23010 hiPSCs: Reprogramming towards cell-based therapies Phillip E. Woolwine Independent Author; phillipwoolwine@gmail.com Received 6 May 2013; revised 13 June 2013; accepted 15 July 2013 Copyright © 2013 Phillip E. Woolwine. This is an open access article distributed under the Creative Commons Attribution License, which permits unrestricted use, distribution, and reproduction in any medium, provided the original work is properly cited. ABSTRACT Stem cell therapies show great potential for use in regenerative medicine, though advancements in safe stem cell technology need to be realized. Human induced pluripotent stem cells (hiPSCs) hold an advantage over other stem cell types for use in cell-based therapies due to their potential as an unlimited source of rejuvenated and im- munocompatible SCs which do not elicit the ethical and moral debates associated with the destruction of human embryos. Towards reali- zation of this potential this review focuses on the recent progress in DNA- and xeno-free re- programming methods, particularly small mole- cule methods, as well as addresses some of the latest insights on donor cell gene expression, telomere dynamics, and epigenetic aberrations that are a potential barrier to successful wide- spread clinical applications. Keywords: iPSC; Reprogramming; Small Molecules; Oct4; Epigenetics; Regenerative Medicine; Cli nica l Reg ulatio ns 1. INTRODUCTION The promise of stem cell-based rejuvenation therapy has long been heralded and has recently been previewed with interim reports of success by companies such as StemCell, Inc. In a Phase I/II trial using their proprietary human neural stem cells (HuCNS-SCs), StemCell, Inc. was able to show allogeneic SCs could improve the sen- sory function of chest-level complete spinal cord injuries [1]. Thus far the results have shown the treatment to be safe owing to the low immunogenicity of fetally-derived SCs, though the patients remain temporarily immuno- suppressed. In another Phase I study these same alloge- neic HuCNS-SCs were shown safe for injection in hu- man brains suffering from Palizaeus-Merzbacher disease (PMD) [2]. There is currently no cure for PMD and chil- dren normally die of this disease around ages 10 - 15 due to severe neurological dysfunction owing to defective oligodendrocytes which fail to myelinate axons (review- ed in [3]). With cautious optimism, early results show that within nine months after the procedure transplanted regions had increased myelination and neurological func- tion. These reports support the great expectations of animal- based SC research translated into human therapies [4]; however, fetal SCs have limited availability and the use of these and related human embryonic SCs (hESCs) still carry many ethical considerations as harvesting these viable embryos destroys potential life [5]. Moreover, though ESCs have low immunogenicity due to their im- muno-suppressive capacity and do not readily provoke a T-cell response, as they differentiate and express more MHC molecules on their surface allogeneic ESCs can provoke an immune response [6] that could necessitate lifelong immuno-suppression o r even make the cond ition worse. While an immunocompatible and potentially unlimited source of autologous hESCs for cell therapy can be derived through somatic cell nuclear transfer (SCNT), hESC generation by SCNT is technically chal- lenging [7] and not only elicits the same ethical debate concerning the destruction of a viable embryo but also that of human cloning—globally banned by the United Nations [8,9]. Though therapeutic cloning is permitted in some countries and US states such as California and Massachusetts [10], public perception and political cli- mates restrict funding for development of this technology. Adult mesenchymal stem cells (MSCs) do not have the ethical or moral complications associated with ESCs, are more readily available, and have already shown efficacy in cell therapy trials to treat myocardial infarction, dia- betes, bone lesions, cartilage damage, and skin burns, among others [11]. However, while MSCs have been shown to have low immunogenicity due to secretion of immune modulators such as IL-10, HLA-G5, and TGFβ1, allogeneic MSCs can become immunogenic upon termi- Copyright © 2013 SciRes. OPEN A CCESS
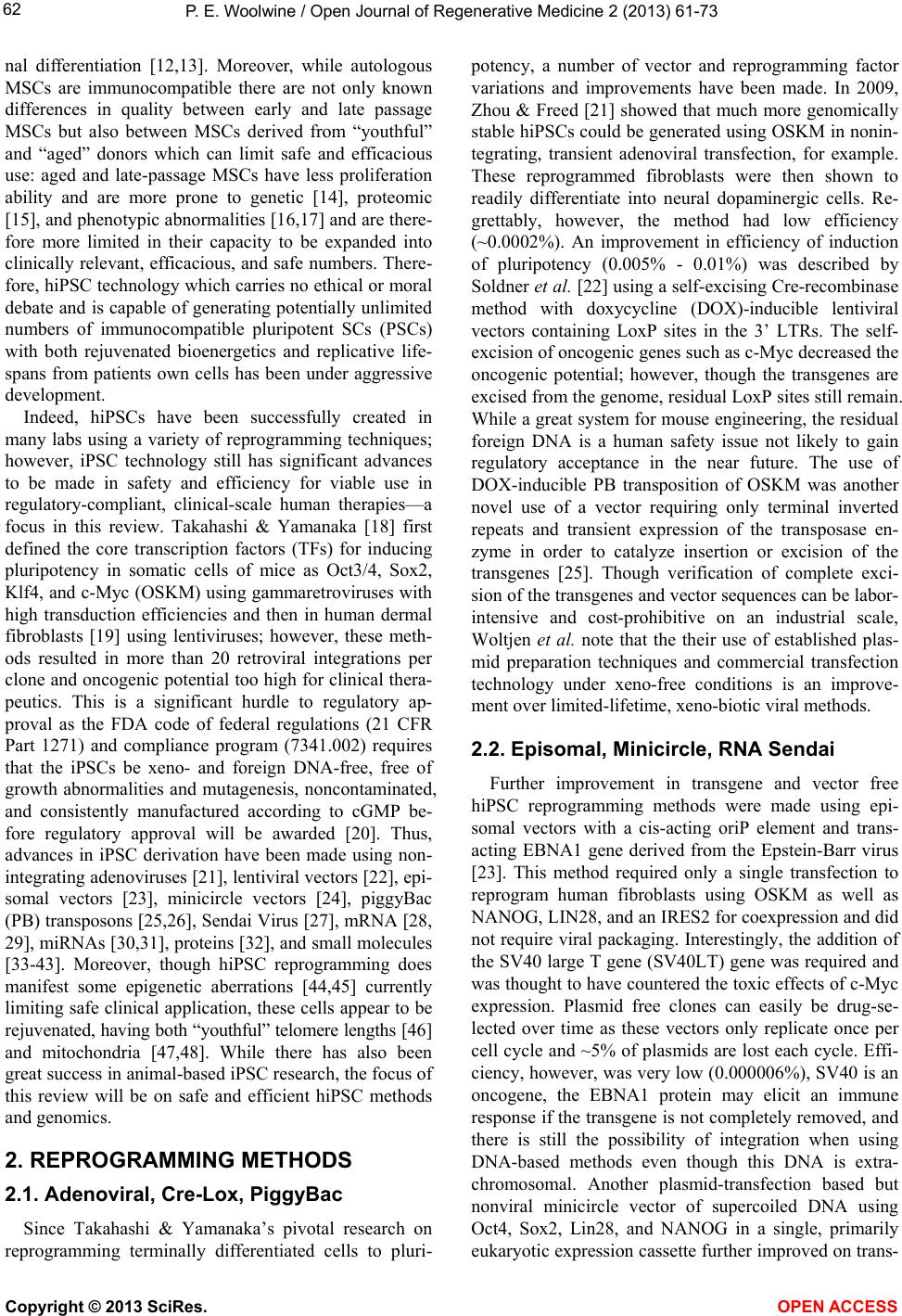 P. E. Woolwine / Open Journal of Rege nerative Medicine 2 (2013) 61-73 62 nal differentiation [12,13]. Moreover, while autologous MSCs are immunocompatible there are not only known differences in quality between early and late passage MSCs but also between MSCs derived from “youthful” and “aged” donors which can limit safe and efficacious use: aged and late-passage MSCs have less proliferation ability and are more prone to genetic [14], proteomic [15], and phenotypic abnormalities [1 6,17] and are th ere- fore more limited in their capacity to be expanded into clinically relevant, efficacious, and safe numbers. There- fore, hiPSC technology which carries no ethical or moral debate and is capable of generating potentially unlimited numbers of immunocompatible pluripotent SCs (PSCs) with both rejuvenated bioenergetics and replicative life- spans from patients own cells has been under aggressive development. Indeed, hiPSCs have been successfully created in many labs using a variety of reprogramming techniques; however, iPSC technology still has significant advances to be made in safety and efficiency for viable use in regulatory-compliant, clinical-scale human therapies—a focus in this review. Takahashi & Yamanaka [18] first defined the core transcription factors (TFs) for inducing pluripotency in somatic cells of mice as Oct3/4, Sox2, Klf4, and c-Myc (OSKM) using gammaretroviruses with high transduction efficiencies and then in human dermal fibroblasts [19] using lentiviruses; however, these meth- ods resulted in more than 20 retroviral integrations per clone and oncogenic po tential too high for clinical thera- peutics. This is a significant hurdle to regulatory ap- proval as the FDA code of federal regulations (21 CFR Part 1271) and compliance program (7341.002) requires that the iPSCs be xeno- and foreign DNA-free, free of growth abnormalities and mutagenesis, noncontaminated, and consistently manufactured according to cGMP be- fore regulatory approval will be awarded [20]. Thus, advances in iPSC derivation have been made using non- integrating ad enoviru ses [21], len tiviral vector s [22], epi- somal vectors [23], minicircle vectors [24], piggyBac (PB) transposons [25,26], Sendai Virus [27], mRNA [28, 29], miRNAs [30,31], proteins [32], and small molecules [33-43]. Moreover, though hiPSC reprogramming does manifest some epigenetic aberrations [44,45] currently limiting safe clinical ap plication, these cells appear to be rej uven ated, having both “youthful” telomere lengths [4 6] and mitochondria [47,48]. While there has also been great success in animal-based iPSC research, the focus of this review will be on safe and efficient hiPSC methods and genomics. 2. REPROGRAMMING METHODS 2.1. Adenoviral, Cre-Lox, PiggyBac Since Takahashi & Yamanaka’s pivotal research on reprogramming terminally differentiated cells to pluri- potency, a number of vector and reprogramming factor variations and improvements have been made. In 2009, Zhou & Freed [21] showed that much more genomically stable hiPSCs could be generated using OSKM in nonin- tegrating, transient adenoviral transfection, for example. These reprogrammed fibroblasts were then shown to readily differentiate into neural dopaminergic cells. Re- grettably, however, the method had low efficiency (~0.0002%). An improvement in efficiency of induction of pluripotency (0.005% - 0.01%) was described by Soldner et al. [22] using a self-excising Cre-recombinase method with doxycycline (DOX)-inducible lentiviral vectors containing LoxP sites in the 3’ LTRs. The self- excision of oncogenic genes such as c-Myc decreased the oncogenic potential; however, though the transgenes are excised from the genome, residual LoxP sites still remain. While a great system for mouse engineering, the residual foreign DNA is a human safety issue not likely to gain regulatory acceptance in the near future. The use of DOX-inducible PB transposition of OSKM was another novel use of a vector requiring only terminal inverted repeats and transient expression of the transposase en- zyme in order to catalyze insertion or excision of the transgenes [25]. Though verification of complete exci- sion of the tr ansgen es and vecto r sequen ces can b e labor- intensive and cost-prohibitive on an industrial scale, Woltjen et al. note that the their use of established plas- mid preparation techniques and commercial transfection technology under xeno-free conditions is an improve- ment over limited-lifetime, xeno-biotic viral methods. 2.2. Episomal, Minicircle, RNA Sendai Further improvement in transgene and vector free hiPSC reprogramming methods were made using epi- somal vectors with a cis-acting oriP element and trans- acting EBNA1 gene derived from the Epstein-Barr virus [23]. This method required only a single transfection to reprogram human fibroblasts using OSKM as well as NANOG, LIN28, and an IRES2 for coexpression and did not require viral packaging. In terestingly, the addition of the SV40 large T gene (SV40LT) gene was required and was thought to have coun tered the toxic effects of c-Myc expression. Plasmid free clones can easily be drug-se- lected over time as these vectors only replicate once per cell cycle and ~5% of plasmids are lost each cycle. Effi- ciency, however, wa s very low (0.000006%) , SV40 is an oncogene, the EBNA1 protein may elicit an immune response if the transgene is not completely removed, and there is still the possibility of integration when using DNA-based methods even though this DNA is extra- chromosomal. Another plasmid-transfection based but nonviral minicircle vector of supercoiled DNA using Oct4, Sox2, Lin28, and NANOG in a single, primarily eukaryotic expression cassette further improved on trans- Copyright © 2013 SciRes. OPEN A CCES S
 P. E. Woolwine / Open Journal of Rege nerative Medicine 2 (2013) 61-73 63 gene and vector free hiPSC reprogramming [24]. Trans- fection efficiencies (~10.8% ± 1.7%) were approx. an order of magnitude higher than with the aforementioned episomal vectors and bene fitted from reduced exogen ous silencing leading to longer ectopic expression; repro- gramming efficiencies were (~0.005%) were also much higher than other transgene and vector-free methods. While this decreased labor associated with reprogram- ming, FDA-required screening for possible DNA inte- gration can still be labor intensive. Improving safety fur- ther, a move towards DNA-free methods without the risk of integration was described by Fusaki et al. [27] using an RNA Sendai virus (SeV) which does not replicate using a DNA phase. Reprogramming efficiencies (1%) using the SeV vector carrying OSKM were much im- proved over retroviral methods. Additionally, the SeV genome is naturally depleted from the cytoplasm by the cell over time. However, SeV can activate innate anti- viral mechanisms; anti-HN protein antibody negative se- lection can easily purify for viral free clones but does add extra labor. 2.3. RNAs Truly xeno-, transgene-, vector sequence-, and DNA- free methods to reprogram human somatic cells without the risk of residual integration using mRNA were first published by Yukabov et al. [28]. Yukabov et al. showed that transfecting in-vitro produced mRNA coding Oct4, Sox2, Lin28, and NANOG could successfully induce human fibroblasts to pluripotency, albeit with low effi- ciency (0.0005%). While RNA reprogramming methods have been known for some time, innate immune re- sponses such as those effected by the RNA helicase reti- noic acid inducible gene I (RIG-I) which detects viral RNA [49] have generally limited success. Research by Angel & Yanik [50] first showed that knockdown of in- nate immune system-related genes such as Ifnb1 and Eif2ak2 using a standard siRNA cocktail could suppress this response and improve RNA delivery methods. Fur- ther advancements were made using synthetic mRNA developed by Warren et al. [29] and delivered using RNAiMAX (Invitrogen) cationic lipid delivery vehicles. Knowing that ssRNA can activate cellular anti-viral mechanisms the authors modified the mRNA by remov- ing the 5’ phosphates with phosphatases; this attenuated interferon signaling. Additionally, by mimicking normal mRNA editing through substitution of either 5-methyl- cytidine (5mC) for cytidine or pseudouridine (psi) for uridine, increased transcript and cell viability was ob- served. The addition of a type I interferon receptor decoy receptor improved viability further. This combination greatly decreased the innate antiviral responses and ini- tial toxicity normally encountered when using unedited RNA. Under these conditions, reprogramming human somatic cells using OSKM as well as Lin28 mRNAs resulted in much improved efficiencies of 1.4% versus 0.04% using retroviral methods; timeframes for deriva- tion (16 days) were approximately half that using retro- viral methods. However, Warren et al. noted that the mRNA reprogramming required daily (16) transfections in order to maintain high levels of ectopic expression, though some reported optimizations in reprogramming factor cocktails and the use of an Oct4-MyoD fusion protein mRNA are capable of reducing required daily transfections to approximately 1 week [51]. Indeed, moderation of innate immune activities may benefit the use of directly transfected mature double- stranded miRNAs such as mir-200 c, mir-302 s, and mir- 369 which induce reprogramming by global demethyla- tion, indirectly promoting expression of pluripotency TFs Oct4 and Sox2 [30,31], for example. miRNAs have shown feasibility in reprogramming human cells but ef- ficiency has suffered, presumably due to detection by RIG-I. While these methods present a much more viable method capable of meeting the strict requirements for safety and regulatory approval while being commercially feasible, the industrial production of modified RNAs could increase costs considerably. 2.4. Directed Delivery of Proteins Employing bioprocesses already in place for the pro- duction of recombinant proteins may improve the com- mercialization of hiPSC technology. Such methods would also bypass the inherent risks of DNA-based ge- nome manipulation and the added complexity of RNA- based methods. It has already been shown feasible that the direct reprogramming of human somatic cells with OSKM proteins is possible, for example, as performed by Kim et al. [32], but with low efficiency (0.001%). A major hurdle to improving protein-based methods is the ability to deliver them across the cell membranes; Kim et al. have made progress in overcoming this hurdle by taking advantage of the viral HIV transactivator of tran- scription (TAT) protein containing a high proportion of basic amino acids known as cell penetrating peptides (CPPs). These CPPs are capable of efficiently entering the cell and nucleus—a quality Kim et al. exploited by anchoring them to OSKM proteins. Further advances in protein-based methods have aimed at stabilizing the pro- tein in culture and improving endosomal release upon uptake. In an optimization of direct protein delivery methods, Their et al. [52] employed media containing KnockOut D-MEM medium supplemented with 2% fetal calf serum, 7.5% serum replacement, and 2.5% lipid rich Albumax to confer enhanced protein stability and trans- duction efficiencies. This media supported recombinant Oct4-TAT reprogramming efficiencies only slightly re- duced and on the same order of magnitude as viral meth- Copyright © 2013 SciRes. OPEN A CCES S
 P. E. Woolwine / Open Journal of Rege nerative Medicine 2 (2013) 61-73 64 ods using transduction of the Oct4 gene. Using similar media, Their et al. [53] have shown recombinant Sox2- TAT is reprogramming competent, though getting the proteins delivered to the right parts of the cell for effi- cient expression needs improvement. 2.5. Small Molecules The use of small molecules represents an advanta- geous approach to consistent and safe derivation of hiPSCs: using small molecules not only circumvents the need for laborious assays proving the absence of adventi- tious agents and/or foreign genetic elements required for FDA approval, particularly if the molecules are already part of an FDA-approved drug library, but also takes advantage of existing industrial drug development infra- structure. Moreover, small molecule platforms are ame- nable to clinical- and industrial-scale high-throughput (HT) platforms that not only include automated cell cul- ture systems such as the CompacT SelecT (TAP Biosys- tems) used by StemCell, Inc. to manufacture their line of HuCNS-SCs for human cell therapy [54] but also HT, label-free microfluidic platforms capable of separating hiPSCs based on their unique adhesion signature [55]— greatly reducing labor while increasing reliability and regulatory standardization. Many compounds which in- crease hiPSC reprogramming efficiency such as through reduced extrinsic and intrinsic apoptosis and modulation of master TFs involved in pluripotency have already been identified, for example. Inh ibition of Caspase3-mediated Rho kinases (ROCKs) which mediate caspase cascades, cell detachment, mem- brane blebbing, and nuclear fragmentation have shown beneficial to cell survival [56]. Thiazovivin, a ROCK inhibitor, is just one example of a small molecule which increases hiPSC survival and colony derivation after cell- cell and cell-substratum detachment d uring sp litting [3 9]. Vitamin C (Vc) is a cheap, readily available, and FDA approved molecule which has also been shown to in- crease survival of viral OSKM reprogrammed somatic (fibroblast) cells [33], for example. Here, Estaben et al. showed Vc could decrease senescence of hiPSC through suppression of p53, research that is supported by [57] who have shown that suppression of the p53-p21 path- way increases virally-based generation of hiPSCs. The exact mechanisms of Vc in cell reprogramming were further elucidated by Wang et al. [41] who showed that Vc promotes the histone demethylase (HDM) activities of Jhdm1a/1b in Oct4 transduced mouse fibroblasts; Jhdm1a/1b (kdm2a/b) not only demethylated H3K36- me2/3 marks in the Ink4/Arf locus—repressing it and senescence while promoting cell cycle progression—but also activated miRs 302/367 in conjunction with Oct4. Estaben et al’s hypothesis that Vc can modulate dioxy- genase hypoxia inducible factor (HIF) target genes as a cofactor for HIF reactions is supported in research by Yoshida et al. [58] whom had first shown that hypoxic (5% O2) culture conditions could improve hiPSC repro- gramming efficiency; HIF-2α is known to directly bind hypoxia response elements (HREs) located in Oct4 pro- moter but also the conserved regions 3 & 4 of the Oct4 distal enhancers (DE) known to drive expression in the ICM and in ES cells [59]. Building on this discovery, a small molecule activator of 3’-phosphoinositide-depen- dent kinase-1 (PDK1) discovered by Zhu et al. [60], PS48, has been shown to activate Akt and stimulate a transition from aerobic to glycolytic metabolism. This transition to anaerobic metabolism is also hypothesized to improve reprogramming through a reduction in mito- chondrial oxidation-associated ROS. Supporting research on this change in bioenergetics, mt morphology and en- ergetics analysis [47,48] have shown that the cristae and energetic capacity of hiPSCs mts undergo a transition and are rejuvenated to a youthful ESC-like state during reprogramming. The addition of the glycolytic interme- diate L-lactate can improve this mt metabolic shift and reprogramming efficiency [36]. Small molecule modulation or replacement of master TFs involved in pluripotency has also been shown to benefit iPSC reprogramming efficiencies. While not shown in human iPSCs, Ichida et al. [34] first showed that Sox2 and C-Myc can be replaced by a small mole- cule inhibitor of TGF-β signaling (E-616452, RepSox) through induction of Nanog in retrovirally reprogram- med mouse embryonic fibroblasts (MEFs). Other re- search using commercially available small molecule in- hibitors of the pan-Src family kinase (SFK) have also shown that activation of Nanog can replace Sox2 to virally reprogram MEFs [61]; SFk inhibitors have not been adequately assayed in hiPSC reprogramming but have been shown to promote epithelial differentiation in hESCs, however [62]. Moreover, it has been shown that removal of C-Myc can increase OKS lentiviral hiPSC reprogramming efficiency in combination with other small molecule inhibitors of TGF-β receptor kinase (SB 431542) as well as inhibitors of MEK signaling (PD 0325901), GSK3β signaling (CHIR 99021), and ROCK inhibition with Thiazov ivin [40]. Indeed, Valamehr et al. showed that using Thiazovivin to decrease apoptosis in combination with small molecules that inhibit TGF-β, MEK, and GSK3β signaling increases hiPSC reprogram- ming efficiency over methods without Thiazovivin-me- diated ROCK inhibition or just ROCK inhibition alone. Interestingly, lithium (Li) was found to be able to replace some core factors in O alone, OK, and OS transduced hiPSCs through partial inhibition of GSK3β signaling, increased transcriptional activity of Nanog, and through inhibition of the H3K4 HDM Kdm1a (LSD1) [42]. However, complete replacement of all reprogramming Copyright © 2013 SciRes. OPEN A CCES S
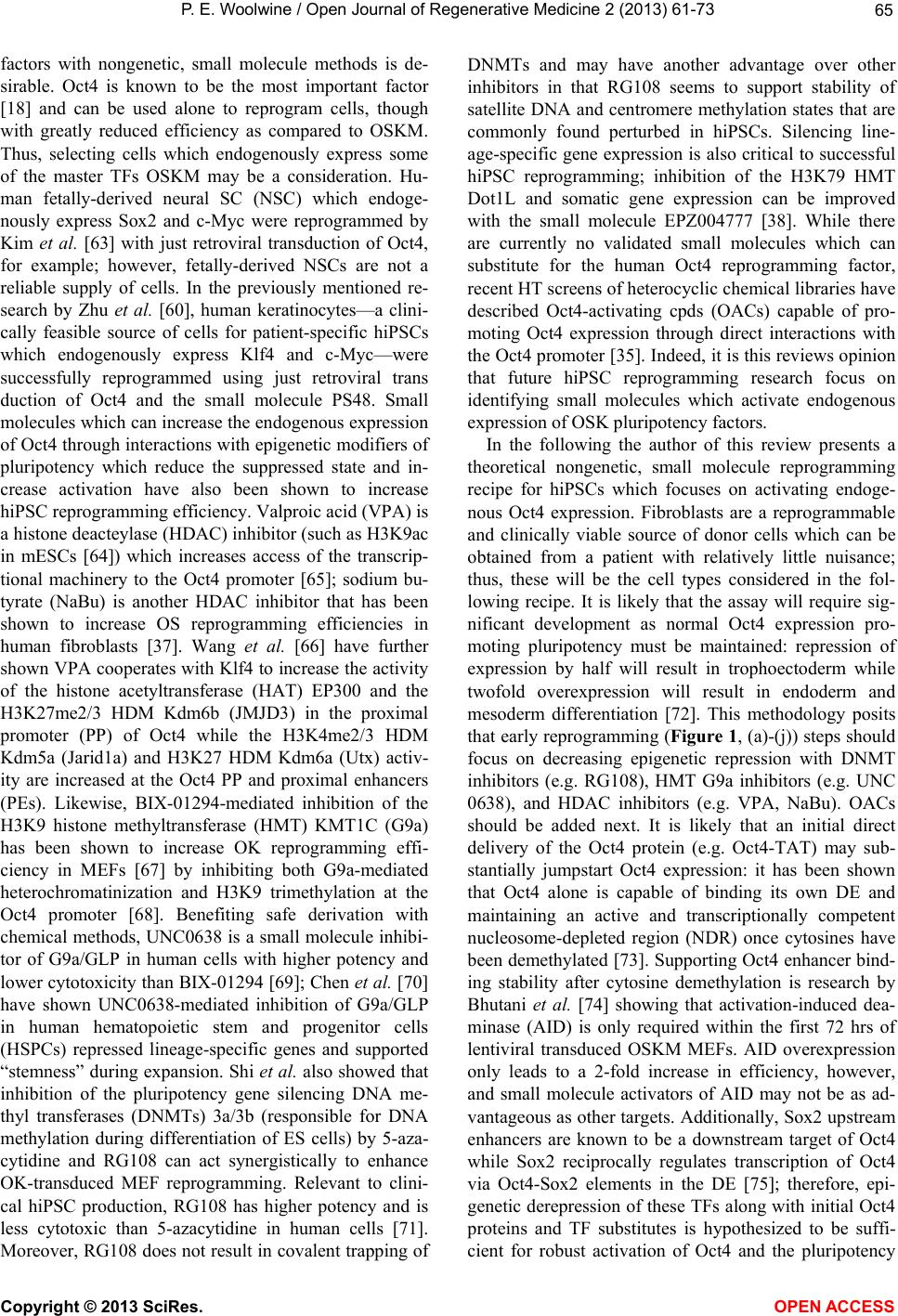 P. E. Woolwine / Open Journal of Rege nerative Medicine 2 (2013) 61-73 65 factors with nongenetic, small molecule methods is de- sirable. Oct4 is known to be the most important factor [18] and can be used alone to reprogram cells, though with greatly reduced efficiency as compared to OSKM. Thus, selecting cells which endogenously express some of the master TFs OSKM may be a consideration. Hu- man fetally-derived neural SC (NSC) which endoge- nously express Sox2 and c-Myc were reprogrammed by Kim et al. [63] with just retroviral transduction of Oct4, for example; however, fetally-derived NSCs are not a reliable supply of cells. In the previously mentioned re- search by Zhu et al. [60], human keratinocytes—a clini- cally feasible source of cells for patient-specific hiPSCs which endogenously express Klf4 and c-Myc—were successfully reprogrammed using just retroviral trans duction of Oct4 and the small molecule PS48. Small molecules which can increase the endogenous expression of Oct4 through interactions with epigenetic modifier s of pluripotency which reduce the suppressed state and in- crease activation have also been shown to increase hiPSC reprogramming efficiency. Valproic acid (VPA) is a histone deacteylase (HDAC) inhibitor (such as H3K9ac in mESCs [64]) which increases access of the transcrip- tional machinery to the Oct4 promoter [65]; sodium bu- tyrate (NaBu) is another HDAC inhibitor that has been shown to increase OS reprogramming efficiencies in human fibroblasts [37]. Wang et al. [66] have further shown VPA cooperates with Klf4 to increase the activity of the histone acetyltransferase (HAT) EP300 and the H3K27me2/3 HDM Kdm6b (JMJD3) in the proximal promoter (PP) of Oct4 while the H3K4me2/3 HDM Kdm5a (Jarid1a) and H3K27 HDM Kdm6a (Utx) activ- ity are increased at the Oct4 PP and proximal enhancers (PEs). Likewise, BIX-01294-mediated inhibition of the H3K9 histone methyltransferase (HMT) KMT1C (G9a) has been shown to increase OK reprogramming effi- ciency in MEFs [67] by inhibiting both G9a-mediated heterochromatinization and H3K9 trimethylation at the Oct4 promoter [68]. Benefiting safe derivation with chemical methods, UNC0638 is a small molecule inhibi- tor of G9a/GLP in human cells with higher potency and lower cytotoxicity than BIX-0129 4 [69]; Chen et al. [70] have shown UNC0638-mediated inhibition of G9a/GLP in human hematopoietic stem and progenitor cells (HSPCs) repressed lineage-specific genes and supported “stemness” during expansion. Shi et al. also showed that inhibition of the pluripotency gene silencing DNA me- thyl transferases (DNMTs) 3a/3b (responsible for DNA methylation during differentiation of ES cells) by 5-aza- cytidine and RG108 can act synergistically to enhance OK-transduced MEF reprogramming. Relevant to clini- cal hiPSC production, RG108 has higher potency and is less cytotoxic than 5-azacytidine in human cells [71]. Moreover, RG108 does not result in covalent trapping of DNMTs and may have another advantage over other inhibitors in that RG108 seems to support stability of satellite DNA and centromere methylation states that are commonly found perturbed in hiPSCs. Silencing line- age-specific gene expression is also critical to successful hiPSC reprogramming; inhibition of the H3K79 HMT Dot1L and somatic gene expression can be improved with the small molecule EPZ004777 [38]. While there are currently no validated small molecules which can substitute for the human Oct4 reprogramming factor, recent HT screens of heterocyclic chemical libraries have described Oct4-activating cpds (OACs) capable of pro- moting Oct4 expression through direct interactions with the Oct4 promoter [35]. Indeed, it is this reviews opinion that future hiPSC reprogramming research focus on identifying small molecules which activate endogenous expression of OSK pl uripotency factor s. In the following the author of this review presents a theoretical nongenetic, small molecule reprogramming recipe for hiPSCs which focuses on activating endoge- nous Oct4 expression. Fibroblasts are a reprogrammable and clinically viable source of donor cells which can be obtained from a patient with relatively little nuisance; thus, these will be the cell types considered in the fol- lowing recipe. It is likely that the assay will require sig- nificant development as normal Oct4 expression pro- moting pluripotency must be maintained: repression of expression by half will result in trophoectoderm while twofold overexpression will result in endoderm and mesoderm differentiation [72]. This methodology posits that early reprogramming (Figure 1, (a)-(j)) steps should focus on decreasing epigenetic repression with DNMT inhibitors (e.g. RG108), HMT G9a inhibitors (e.g. UNC 0638), and HDAC inhibitors (e.g. VPA, NaBu). OACs should be added next. It is likely that an initial direct delivery of the Oct4 protein (e.g. Oct4-TAT) may sub- stantially jumpstart Oct4 expression: it has been shown that Oct4 alone is capable of binding its own DE and maintaining an active and transcriptionally competent nucleosome-depleted region (NDR) once cytosines have been demethylated [73]. Supporting Oct4 enhancer bind- ing stability after cytosine demethylation is research by Bhutani et al. [74] showing that activation-induced dea- minase (AID) is only required within the first 72 hrs of lentiviral transduced OSKM MEFs. AID overexpression only leads to a 2-fold increase in efficiency, however, and small molecule activators of AID may not be as ad- vantageous as other targets. Additionally, Sox2 upstream enhancers are known to be a downstream target of Oct4 while Sox2 reciprocally regulates transcription of Oct4 via Oct4-Sox2 elements in the DE [75]; therefore, epi- genetic derepression of these TFs along with initial Oct4 proteins and TF substitutes is hypothesized to be suffi- cient for robust activation of Oct4 and the pluripotency Copyright © 2013 SciRes. OPEN A CCES S
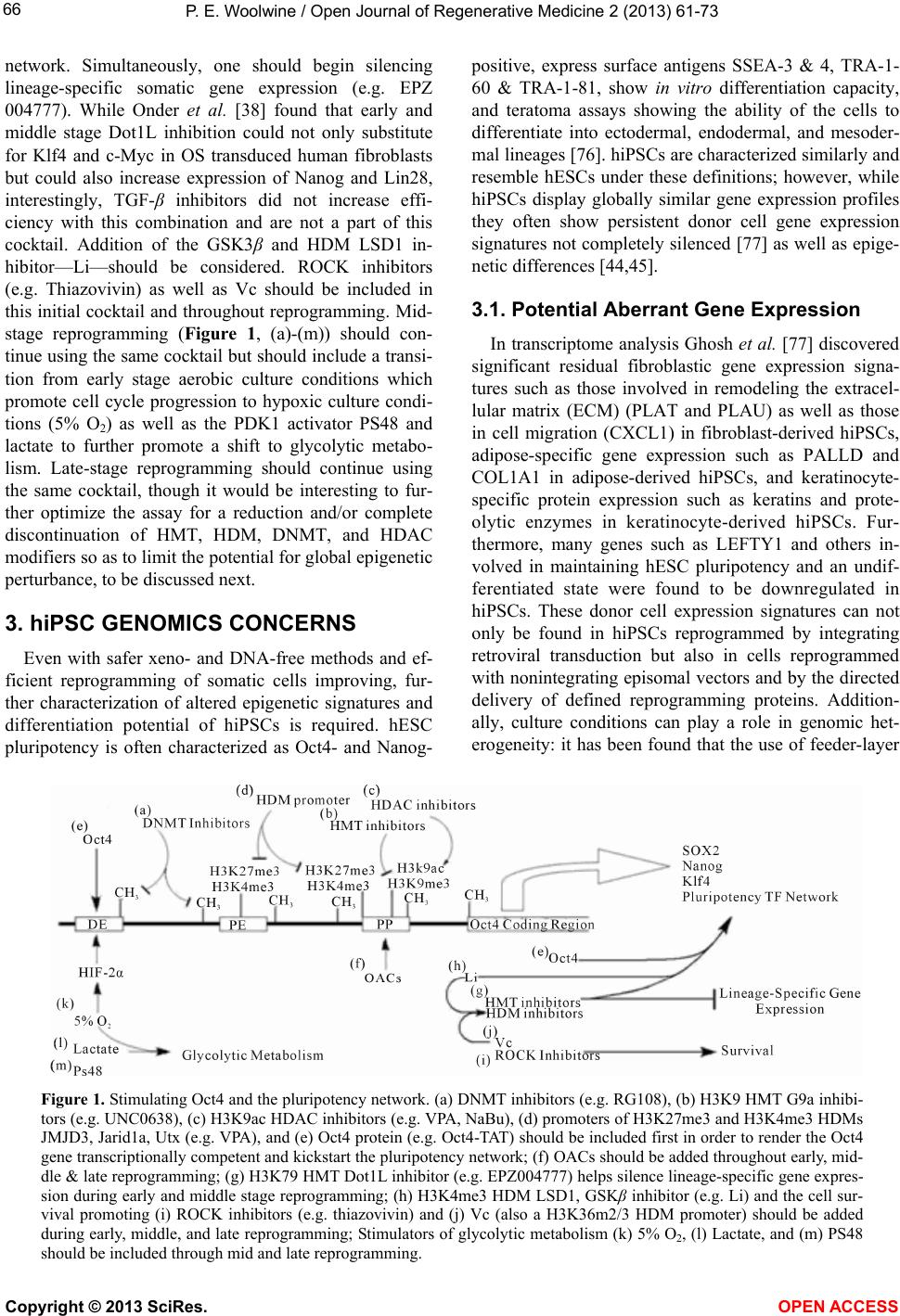 P. E. Woolwine / Open Journal of Rege nerative Medicine 2 (2013) 61-73 Copyright © 2013 SciRes. 66 positive, express surface antigens SSEA-3 & 4, TRA-1- 60 & TRA-1-81, show in vitro differentiation capacity, and teratoma assays showing the ability of the cells to differentiate into ectodermal, endodermal, and mesoder- mal lineages [76]. hiPSCs are characterized similarly and resemble hESCs under these definitions; however, while hiPSCs display globally similar gene expression profiles they often show persistent donor cell gene expression signatures not completely silenced [77] as well as epige- netic differ ences [44,45]. network. Simultaneously, one should begin silencing lineage-specific somatic gene expression (e.g. EPZ 004777). While Onder et al. [38] found that early and middle stage Dot1L inhibition could not only substitute for Klf4 and c-Myc in OS transduced human fibroblasts but could also increase expression of Nanog and Lin28, interestingly, TGF-β inhibitors did not increase effi- ciency with this combination and are not a part of this cocktail. Addition of the GSK3β and HDM LSD1 in- hibitor—Li—should be considered. ROCK inhibitors (e.g. Thiazovivin) as well as Vc should be included in this initial cocktail an d throughou t reprogramming. Mid- stage reprogramming (Figure 1, (a)-(m)) should con- tinue using the same cock tail but should include a transi- tion from early stage aerobic culture conditions which promote cell cycle progression to hypoxic culture condi- tions (5% O2) as well as the PDK1 activator PS48 and lactate to further promote a shift to glycolytic metabo- lism. Late-stage reprogramming should continue using the same cocktail, though it would be interesting to fur- ther optimize the assay for a reduction and/or complete discontinuation of HMT, HDM, DNMT, and HDAC modifiers so as to limit the potential for global epigen etic perturbance, to be discussed next. 3.1. Potential Aberrant Gene Expression In transcriptome analysis Ghosh et al. [77] discovered significant residual fibroblastic gene expression signa- tures such as those involved in remodeling the extracel- lular matrix (ECM) (PLAT and PLAU) as well as those in cell migration (CXCL1) in fibroblast-derived hiPSCs, adipose-specific gene expression such as PALLD and COL1A1 in adipose-derived hiPSCs, and keratinocyte- specific protein expression such as keratins and prote- olytic enzymes in keratinocyte-derived hiPSCs. Fur- thermore, many genes such as LEFTY1 and others in- volved in maintaining hESC pluripotency and an undif- ferentiated state were found to be downregulated in hiPSCs. These donor cell expression signatures can not only be found in hiPSCs reprogrammed by integrating retroviral transduction but also in cells reprogrammed with nonintegrating episomal vectors and by the directed delivery of defined reprogramming proteins. Addition- ally, culture conditions can play a role in genomic het- erogeneity: it has been found that the use of feeder-layer 3. hiPSC GENOMICS CONCERNS Even with safer xeno- and DNA-free methods and ef- ficient reprogramming of somatic cells improving, fur- ther characterization of altered epigenetic signatures and differentiation potential of hiPSCs is required. hESC pluripotency is often characterized as Oct4- and Nanog- Figure 1. Stimulating Oct4 and the pluripotency network. (a) DNMT inhibitors (e.g. RG108), (b) H3K9 HMT G9a inhibi- tors (e.g. UNC0638), (c) H3K9ac HDAC inhibitors (e.g. VPA, NaBu), (d) promoters of H3K27me3 and H3K4me3 HDMs JMJD3, Jarid1a, Utx (e.g. VPA), and (e) Oct4 protein (e.g. Oct4-TAT) should be included first in order to render the Oct4 gene transcriptionally competent and kickstart the pluripotency network; (f) OACs should be added throughout early, mid- dle & late reprogramming; (g) H3K79 HMT Dot1L inhibitor (e.g. EPZ004777) helps silence lineage-specific gene expres- sion during early and middle stage reprogramming; (h) H3K4me3 HDM LSD1, GSKβ inhibitor (e.g. Li) and the cell sur- vival promoting (i) ROCK inhibitors (e.g. thiazovivin) and (j) Vc (also a H3K36m2/3 HDM promoter) should be added during early, middle, and late reprogramming; Stimulators of glycolytic metabolism (k) 5% O2, (l) Lactate, and (m) PS48 should be included through mid and late reprogramming. OPEN A CCESS
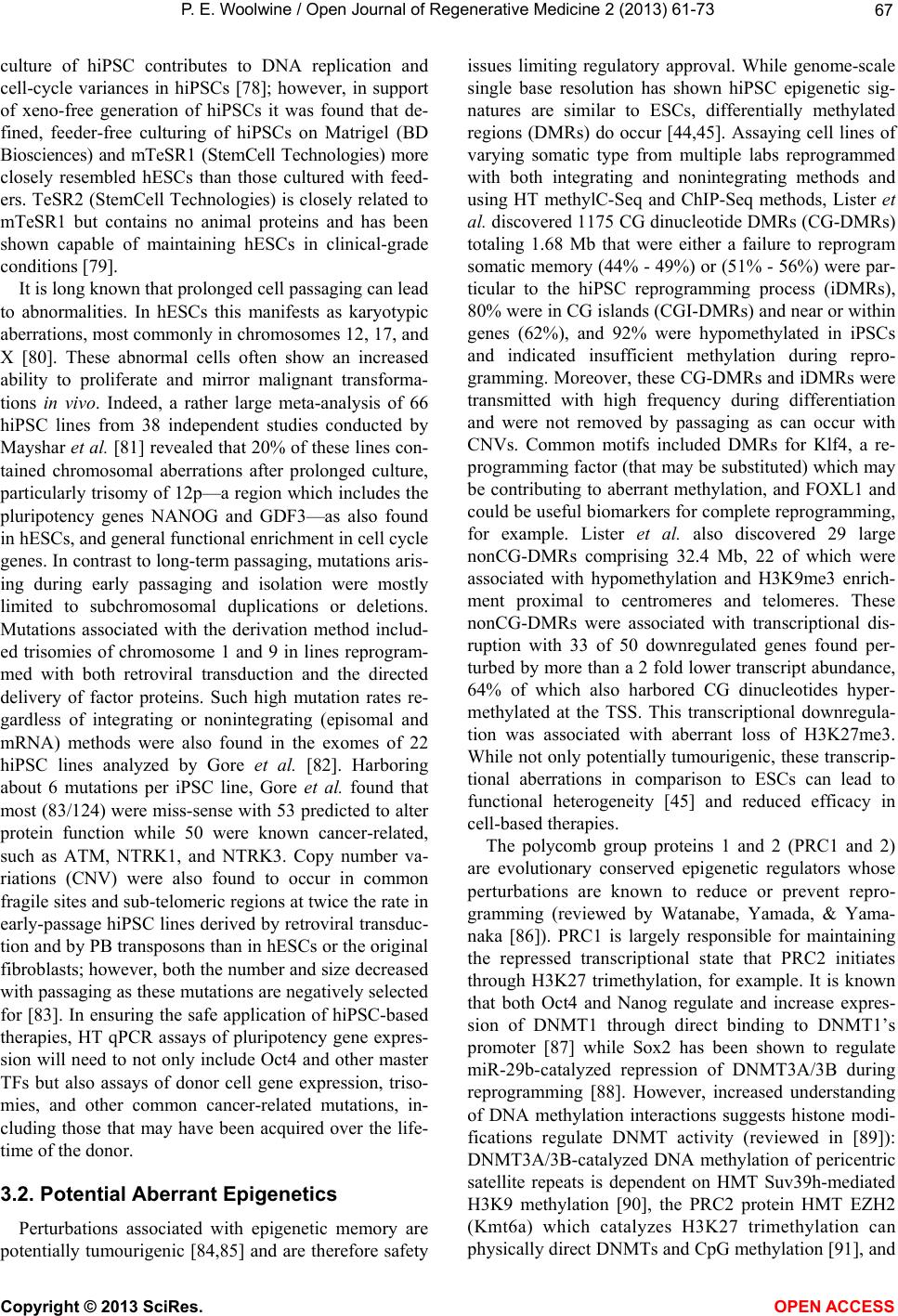 P. E. Woolwine / Open Journal of Rege nerative Medicine 2 (2013) 61-73 67 culture of hiPSC contributes to DNA replication and cell-cycle variances in hiPSCs [78]; however, in support of xeno-free generation of hiPSCs it was found that de- fined, feeder-free culturing of hiPSCs on Matrigel (BD Biosciences) and mTeSR1 (StemCell Technologies) more closely resembled hESCs than those cultured with feed- ers. TeSR2 (StemCell Technologies) is closely related to mTeSR1 but contains no animal proteins and has been shown capable of maintaining hESCs in clinical-grade conditions [79]. It is long known that prolonged cell passaging can lead to abnormalities. In hESCs this manifests as karyotypic aberrations, most commonly in chromosomes 12, 17, and X [80]. These abnormal cells often show an increased ability to proliferate and mirror malignant transforma- tions in vivo. Indeed, a rather large meta-analysis of 66 hiPSC lines from 38 independent studies conducted by Mayshar et al. [81] revealed that 20% of these lines con- tained chromosomal aberrations after prolonged culture, particularly trisomy of 12p—a region which includes the pluripotency genes NANOG and GDF3—as also found in hESCs, and general functional enrich ment in cell cycle genes. In contrast to long-term passaging, mutations aris- ing during early passaging and isolation were mostly limited to subchromosomal duplications or deletions. Mutations associated with the derivation method includ- ed trisomies of chromosome 1 and 9 in lines reprogram- med with both retroviral transduction and the directed delivery of factor proteins. Such high mutation rates re- gardless of integrating or nonintegrating (episomal and mRNA) methods were also found in the exomes of 22 hiPSC lines analyzed by Gore et al. [82]. Harboring about 6 mutations per iPSC line, Gore et al. found that most (83/124) were miss-sense with 53 predicted to alter protein function while 50 were known cancer-related, such as ATM, NTRK1, and NTRK3. Copy number va- riations (CNV) were also found to occur in common fragile sites and sub-telomeric regions at twice the rate in early-passage hiPSC lines derived by retroviral transduc- tion and by PB transposon s than in hESCs or the original fibroblasts; however, both the number and size decreased with passaging as these mutations are neg atively selected for [83]. In ensuring the safe application of hiPSC-based therapies, HT qPCR assays of pluripotency gene expres- sion will need to not only include Oct4 and other master TFs but also assays of donor cell gene expression, triso- mies, and other common cancer-related mutations, in- cluding those that may have been acquired over the life- time of the donor. 3.2. Potential Aberrant Epigenetics Perturbations associated with epigenetic memory are potentially tumourigenic [84,85] and are therefore safety issues limiting regulatory approval. While genome-scale single base resolution has shown hiPSC epigenetic sig- natures are similar to ESCs, differentially methylated regions (DMRs) do occur [44,45]. Assaying cell lines of varying somatic type from multiple labs reprogrammed with both integrating and nonintegrating methods and using HT methylC-Seq and ChIP-Seq methods, Lister et al. discovered 1175 CG dinucleotide DMRs (CG-DMRs) totaling 1.68 Mb that were either a failure to reprogram somatic memory (44% - 49%) or (51% - 56%) were par- ticular to the hiPSC reprogramming process (iDMRs), 80% were in CG islands (CGI-DMRs) and near or within genes (62%), and 92% were hypomethylated in iPSCs and indicated insufficient methylation during repro- gramming. Moreover, these CG-DMRs and iDMRs were transmitted with high frequency during differentiation and were not removed by passaging as can occur with CNVs. Common motifs included DMRs for Klf4, a re- programming factor (that may be substituted) which may be contributing to aberrant methylation, and FOXL1 and could be useful biomarkers for complete reprogramming, for example. Lister et al. also discovered 29 large nonCG-DMRs comprising 32.4 Mb, 22 of which were associated with hypomethylation and H3K9me3 enrich- ment proximal to centromeres and telomeres. These nonCG-DMRs were associated with transcriptional dis- ruption with 33 of 50 downregulated genes found per- turbed by more than a 2 fold lower tran script abundance, 64% of which also harbored CG dinucleotides hyper- methylated at the TSS. This transcriptional downregula- tion was associated with aberrant loss of H3K27me3. While not only po tentially tumourigen ic, these transcrip- tional aberrations in comparison to ESCs can lead to functional heterogeneity [45] and reduced efficacy in cell-based therapies. The polycomb group proteins 1 and 2 (PRC1 and 2) are evolutionary conserved epigenetic regulators whose perturbations are known to reduce or prevent repro- gramming (reviewed by Watanabe, Yamada, & Yama- naka [86]). PRC1 is largely responsible for maintaining the repressed transcriptional state that PRC2 initiates through H3K27 trimethylation, for example. It is known that both Oct4 and Nanog regulate and increase expres- sion of DNMT1 through direct binding to DNMT1’s promoter [87] while Sox2 has been shown to regulate miR-29b-catalyzed repression of DNMT3A/3B during reprogramming [88]. However, increased understanding of DNA methylation interactions suggests histone modi- fications regulate DNMT activity (reviewed in [89]): DNMT3A/3B-catalyzed DNA methylation of pericentric satellite repeats is dependent on HMT Suv39h-mediated H3K9 methylation [90], the PRC2 protein HMT EZH2 (Kmt6a) which catalyzes H3K27 trimethylation can physically direct DNMTs and CpG methylation [91], and Copyright © 2013 SciRes. OPEN A CCES S
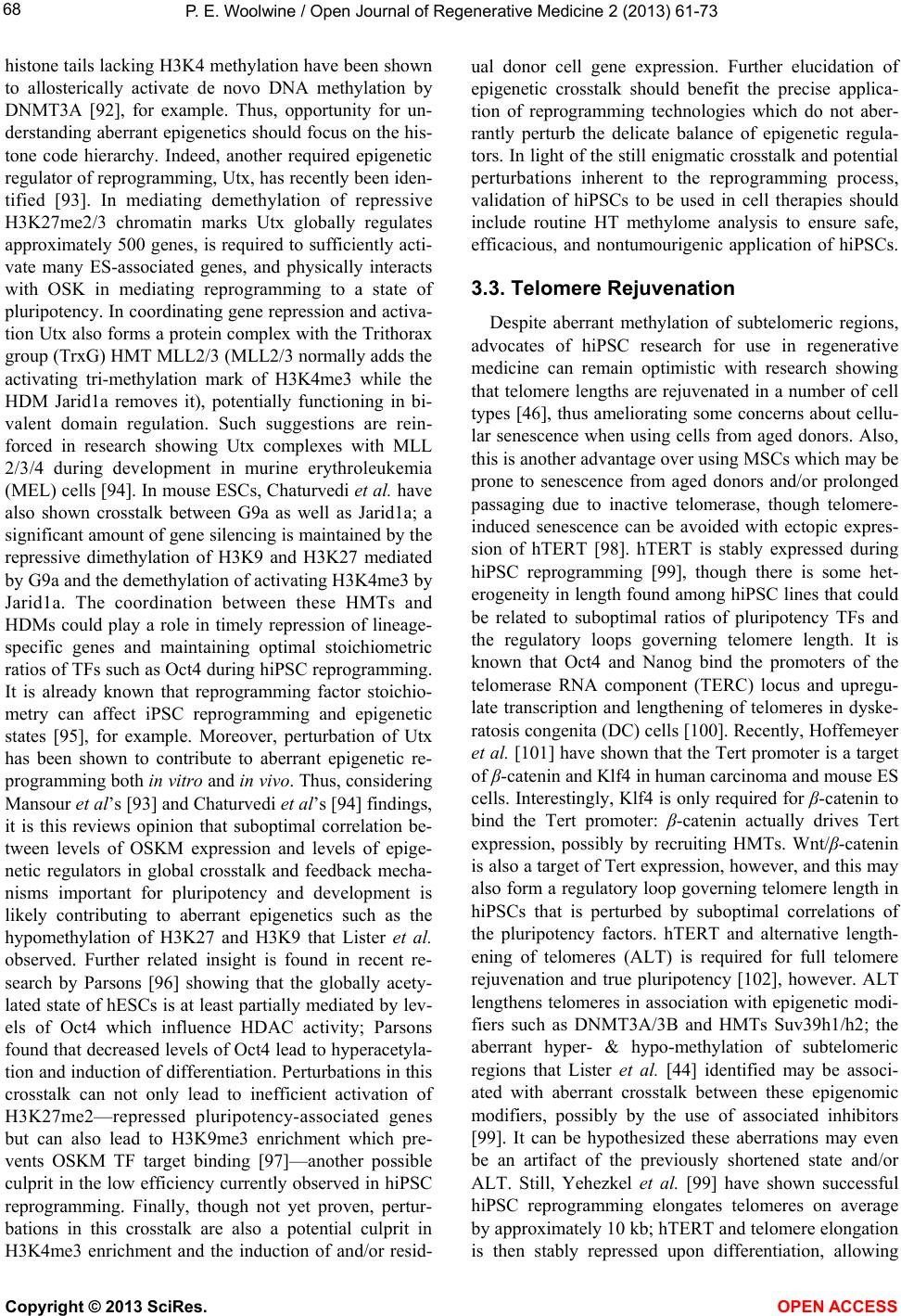 P. E. Woolwine / Open Journal of Rege nerative Medicine 2 (2013) 61-73 68 histone tails lacking H3K4 methylation h ave been shown to allosterically activate de novo DNA methylation by DNMT3A [92], for example. Thus, opportunity for un- derstanding aberrant epigenetics should focus on the his- tone code hierarchy. Indeed, another required epigenetic regulator of reprogramming, Utx, has recently been iden- tified [93]. In mediating demethylation of repressive H3K27me2/3 chromatin marks Utx globally regulates approximately 500 genes, is required to sufficiently acti- vate many ES-associated genes, and physically interacts with OSK in mediating reprogramming to a state of pluripotency. In coordinating gene repression and activa- tion Utx also forms a protein complex with the Trithorax group (TrxG) HMT MLL2/3 (MLL2/3 normally adds the activating tri-methylation mark of H3K4me3 while the HDM Jarid1a removes it), potentially functioning in bi- valent domain regulation. Such suggestions are rein- forced in research showing Utx complexes with MLL 2/3/4 during development in murine erythroleukemia (MEL) cells [94]. In mouse ESCs, Chaturvedi et al. have also shown crosstalk between G9a as well as Jarid1a; a significant amount of gene silencing is maintained by the repressive dimethylation of H3K9 and H3K27 mediated by G9a and the demethylation of activating H3K4me3 by Jarid1a. The coordination between these HMTs and HDMs could play a role in timely repression of lineage- specific genes and maintaining optimal stoichiometric ratios of TFs such as Oct4 during hiPSC reprogramming. It is already known that reprogramming factor stoichio- metry can affect iPSC reprogramming and epigenetic states [95], for example. Moreover, perturbation of Utx has been shown to contribute to aberrant epigenetic re- programming both in vitro and in vivo. Thus, considering Mansour et al’s [93] and Chaturvedi et al’s [94] findings, it is this reviews opinion that suboptimal correlation be- tween levels of OSKM expression and levels of epige- netic regulators in global crosstalk and feedback mecha- nisms important for pluripotency and development is likely contributing to aberrant epigenetics such as the hypomethylation of H3K27 and H3K9 that Lister et al. observed. Further related insight is found in recent re- search by Parsons [96] showing that the globally acety- lated state of hESCs is at least partially mediated b y lev- els of Oct4 which influence HDAC activity; Parsons found that decreased levels of Oct4 lead to hyperacetyla- tion and induction of differentiation. Perturbations in this crosstalk can not only lead to inefficient activation of H3K27me2—repressed pluripotency-associated genes but can also lead to H3K9me3 enrichment which pre- vents OSKM TF target binding [97]—another possible culprit in the low efficien cy currently observed in hiPSC reprogramming. Finally, though not yet proven, pertur- bations in this crosstalk are also a potential culprit in H3K4me3 enrichment and the induction of and/or resid- ual donor cell gene expression. Further elucidation of epigenetic crosstalk should benefit the precise applica- tion of reprogramming technologies which do not aber- rantly perturb the delicate balance of epigenetic regula- tors. In light of the still en igmatic crosstalk and potential perturbations inherent to the reprogramming process, validation of hiPSCs to be used in cell therapies should include routine HT methylome analysis to ensure safe, efficacious, and nontumourigenic application of hiPSCs. 3.3. Telomere Rejuvenation Despite aberrant methylation of subtelomeric regions, advocates of hiPSC research for use in regenerative medicine can remain optimistic with research showing that telomere lengths are rejuvenated in a number of cell types [46], thus ameliorating some concerns about cellu- lar senescence when using cells from aged donors. Also, this is another advantage over using MSCs which may be prone to senescence from aged donors and/or prolonged passaging due to inactive telomerase, though telomere- induced senescence can be avoided with ectopic expres- sion of hTERT [98]. hTERT is stably expressed during hiPSC reprogramming [99], though there is some het- erogeneity in length found among hiPSC lines that could be related to suboptimal ratios of pluripotency TFs and the regulatory loops governing telomere length. It is known that Oct4 and Nanog bind the promoters of the telomerase RNA component (TERC) locus and upregu- late transcription and lengthening of telomeres in dyske- ratosis congenita (DC) cells [100]. Recently, Hoffemeyer et al. [101] have shown that the Tert promoter is a target of β-catenin and Klf4 in human carcinoma and mouse ES cells. Interestingly, Klf4 is only required for β-catenin to bind the Tert promoter: β-catenin actually drives Tert expression, possibly by recruiting HMTs. Wnt/β-catenin is also a target of Tert expression, however, and this may also form a regulatory loop governing telomere length in hiPSCs that is perturbed by suboptimal correlations of the pluripotency factors. hTERT and alternative length- ening of telomeres (ALT) is required for full telomere rejuvenation and true pluripotency [102], however. ALT lengthens telomeres in association with epigenetic modi- fiers such as DNMT3A/3B and HMTs Suv39h1/h2; the aberrant hyper- & hypo-methylation of subtelomeric regions that Lister et al. [44] identified may be associ- ated with aberrant crosstalk between these epigenomic modifiers, possibly by the use of associated inhibitors [99]. It can be hypothesized these aberrations may even be an artifact of the previously shortened state and/or ALT. Still, Yehezkel et al. [99] have shown successful hiPSC reprogramming elongates telomeres on average by approximately 10 kb ; hTERT and telomere elong ation is then stably repressed upon differentiation, allowing Copyright © 2013 SciRes. OPEN A CCES S
 P. E. Woolwine / Open Journal of Rege nerative Medicine 2 (2013) 61-73 69 normal telomere shortening. However, a safe and effica- cious replicative lifespan fo r hiPSCs used in cell therapy must be shown: this review suggests routine telomere assays not only include an assay of hTERT expression but also assays of absolute telomere length such as with modified Cawthon HT qPCR [103]. 4. CONCLUSION SCs for use in cell-based therapies have recently shown interim clinical viability and hold great potential for use in regenerative medicine. There are many regula- tory and biological concerns to be resolved before com- mercialization of SCs for clinical therapy can be achie- ved, however: the use of hESCs in such therapies not only carries great ethical debate but also poses an immu- nogenicity risk; SCNT technology is technically chal- lenging and also controversial; allogeneic MSCs may still pose an immunogenicity risk while autologous MSCs may be susceptible to senescence. In contrast, autologous hiPSCs have all the potential of hESCs and are a source of potentially unlimited, immunocompatible SCs with rejuvenated bioenergetics and replicative lifespans for patient-specific cell-based therapies that pose no ethical dilemma; however, iPSC reprogramming methods still suffer from safety, efficiency, and efficacy concerns, though these obstacles are being surmounted. Since Ta- kahashi & Yamanaka first defined the core OSKM TFs required to reprogram somatic cells to iPSCs using inte- grating viral transduction methods, much progress has been made in developing safer nonintegrating-, RNA-, protein-, and small molecule-based methods. Towards the goal of clinically viable hiPSC technology, strategies exploiting modifiers of cell survival, endogenous pluri- potent TF expression, and epigenetic regulation have been developed to increase hiPSC reprogramming safety, efficiency, and efficacy. However, epigenetic memory of hiPSCs still poses a safety and efficacy concern. This review has discussed the latest discoveries benefiting an increa sed understand ing of plurip otent TF and ep igenetic regulator crosstalk perturbed during reprogramming. This knowledge is paramount to developing reprogram- ming technology which completely silences lineage- specific gene expression, maintains telomere integrity, and circumvents aberrant epigenetic methylation. Per- turbations in hiPSC methylomes may be a result of suboptimal correlations between pluripotency factors and epigenetic regulators during hiPSC reprogramming and more research is needed in this regard. The use of non- genetic small-molecule methods to very precisely restart the endogenous expression of required TFs such as OSK may ameliorate the epigen etic scars of forced OSKM TF expression characteristic of other methods; however, there is currently insufficient genomics data concerning the epigenetic landscape of hiPSCs reprogrammed using still nascent small molecule—only methods to know at this time. Nevertheless, it is this review’s final opinion that DNA- and xeno-free small molecule methods hold the most potential as a clinically viable and relatively lower-cost HT technology capable of generating hiPSCs in a safe, regulatory-compliant, and efficacious platform. REFERENCES [1] StemCells Inc. (2012) Reports positive interim data from spinal cord injury trial. News Rel ease s. http://investor.stemcellsinc.com/phoenix.zhtml?c=86230 &p=irol-newsArticle&ID=1730805&highlight= [2] Gupta, et al. (2012) Neural stem cell engraftment and myelination in the human brain. Science Translational Medicine, 4, 155ra137. doi:10.1126/scitranslmed.3004373 [3] Garbern, J.Y. (2006) Pelizaeus-Merzbacher disease: Ge- netic and cellular pathogenesis. Cellular and Molecular Life Sciences, 64, 50-65. [4] Giusto, E., Donegà, M., Cossetti, C. and Pluchino, S. (2006) Neuro-immune interactions of neural stem cell transplants: From animal disease models to human trials. Experimental Neurology, S0014-4886(13)00092-7. doi:10.1016/j.expneurol.2013.03.009 [5] Lo, B. and Parnham, L. (2009) Ethical issues in stem cell research. Endocrine Reviews, 30, 204-213. doi:10.1210/er.2008-0031 [6] English, K. and Wood, K.J. (2011) Immunogenicity of embryonic stem cell-derived progenitors after transplan- tation. Current Opinion in Organ Transplantation, 16, 90-95. doi:10.1097/MOT.0b013e3283424faa [7] Pan, G., Wang, T., Yao, H. and Pei, D. (2012) Somatic cell reprogramming for regenerative medicine: SCNT vs. iPS cells. Bioessays, 34, 472-476. doi:10.1002/bies.201100174 [8] UN (2005) 59/280—United nations declaration on human cloning. http://daccess-dds-ny.un.org/doc/UNDOC/GEN/N04/493/ 06/PDF/N0449306.pdf?OpenElement [9] Mayor, S. (2005) UN committee approves declaration on human cloning. BMJ, 330, 496. [10] Stabile, B. (2007) Demographic profile of states with human cloning laws: Morality policy meets political eco- nomy. Politics and the Life Sciences, 26, 43-50. doi:10.2990/26_1_43 [11] Wang, S., Qu, X. and Zhao, R.C. (2012a) Clinical appli- cations of mesenchymal stem cells. Journal of Hematol- ogy & Oncology, 5, 19. doi:10.1186/1756-8722-5-19 [12] Griffin, M.D., Ritter, T. and Mahon, B.P. (2010) Immu- nological aspects of allogeneic mesenchymal stem cell therapies. Human Gene Therapy, 21, 1641-1655. doi:10.1089/hum.2010.156 [13] Griffin, M.D., Ryan, A.E., Alagesan, S., Lohan, P., Treacy, O. and Ritter, T. (2013) Anti-donor immune responses elicited by allogeneic mesenchymal stem cells: What have we learned so far? Immunology & Cell Biology, 91, Copyright © 2013 SciRes. OPEN A CCES S
 P. E. Woolwine / Open Journal of Rege nerative Medicine 2 (2013) 61-73 70 40-51. doi:10.1038/icb.2012.67 [14] Choi, M.R., et al. (2012) Genome-scale DNA methylation pattern profiling of human bone marrow mesenchymal stem cells in long-term culture. Experimental & Molecu- lar Medicine, 44, 503-512. doi:10.3858/emm.2012.44.8.057 [15] Capra, E., et al. (2012) Changes in the proteomic profile of adipose tissue-derived mesenchymal stem cells during passages. Proteome Science, 10, 46. doi:10.1186/1477-5956-10-46 [16] Brohlin, M., Kingham, P.J., Novikova, L.N., Novikov, L.N. and Wiberg, M. (2012) Aging effect on neurotrophic activity of human mesenchymal stem cells. PLoS One, 7, e45052. doi:10.1371/journal.pone.0045052 [17] Lohmann, M., et al. (2012) Donor age of human platelet lysate affects proliferation and differentiation of mesen- chymal stem cells. PLoS One, 7, e37839. doi:10.1371/journal.pone.0037839 [18] Takahashi, K. and Yamanaka, S. (2006) Induction of pluripotent stem cells from mouse embryonic and adult fibroblast cultures by defined factors. Cell, 126, 663-676. [19] Yamanaka, et al. (2007) Induction of pluripotent stem cells from adult human fibroblasts by defined factors. Cell, 131, 861-872. [20] FDA (2012) Code of federal regulations title 21, part 1271 human cells, tissues, and cellular and tissue-based products. 66 fr 5466. [21] Zhou, W. and Freed, C.R. (2009) Adenoviral gene deliv- ery can reprogram human fibroblasts to induced pluripo- tent stem cells. Stem Cells, 27, 2667-2674. doi:10.1002/stem.201 [22] Soldner, F., et al. (2009) Parkinson’s disease patient- derived induced pluripotent stem cells free of viral repro- gramming factors. Cell, 136, 964-977. doi:10.1016/j.cell.2009.02.013 [23] Yu, J.Y., et al. (2009) Human induced pluripotent stem cells free of vector and transgene sequences. Science, 324, 797-801.doi:10.1126/science.1172482 [24] Jia, et al. (2010) A nonviral minicircle vector for deriving human iPS cells. Nature Methods, 7, 197-201. doi:10.1038/nmeth.1426 [25] Woltjen, et al. (2009) piggyBac transposition reprograms fibroblasts to induced pluripotent stem cells. Nature, 458, 766-770. doi:10.1038/nature07863 [26] Kaji, K., Norrby, K., Paca, A., Mileikovsky, M., Mohseni, P. and Woltjen, K. (2009) Virus-free induction of pluripo- tency and subsequent excision of reprogramming factors. Nature, 458, 771-775. doi:10.1038/nature07864 [27] Fusaki, N., Ban, H., Nishiyama, A., Saeki, K. and Hase- gawa, M. (2009) Efficient induction of transgene-free human pluripotent stem cells using a vector based on Sendai virus, an RNA virus that does not integrate into the host genome. Proceedings of the Japan Academy. Se- ries B, Physical and Biological Sciences, 85, 348-362. [28] Yakubov, E., Rechavi, G., Rozenblatt, S. and Givol, D. (2010) Reprogramming of human fibroblasts to pluripo- tent stem cells using mRNA of four transcription factors. Biochemical and Biophysical Research Communications, 394, 189-193. doi:10.1016/j.bbrc.2010.02.150 [29] Warren, L., et al. (2010) Highly efficient reprogramming to pluripotency and directed differentiation of human cells with synthetic modified mRNA. Cell Stem Cell, 7, 618-630. doi:10.1016/j.stem.2010.08.012 [30] Miyoshi, N., et al. (2011) Reprogramming of mouse and human cells to pluripotency using mature MicroRNAs. Cell Stem Cell, 8, 633-638. doi:10.1016/j.stem.2011.05.001 [31] Anokye-Danso, F., et al. (2011) Highly efficient miRNA- mediated reprogramming of mouse and human somatic cells to pluripotency. Cell Stem Cell, 8, 376-388. doi:10.1016/j.stem.2011.03.001 [32] Kim, D., et al. (2009) Generation of human induced pluripotent stem cells by direct delivery of reprogram- ming proteins. Cell Stem Cell, 4, 472-476. doi:10.1016/j.stem.2009.05.005 [33] Esteban, M.A., et al. (2010) Vitamin C enhances the gen- eration of mouse and human induced pluripotent stem cells. Cell Stem Cell, 6, 71-79. doi:10.1016/j.stem.2009.12.001 [34] Ichida, J.K., et al. (2009) A small-molecule inhibitor of tgf-B signaling replaces sox2 in reprogramming by in- ducing nanog. Cell Stem Cell, 5, 491-503. doi:10.1016/j.stem.2009.09.012 [35] Li, et al. (2012) Identification of Oct4-activating com- pounds that enhance reprogramming efficiency. Proceed- ings of the National Academy of Sciences, 109, 20853- 20858. doi:10.1073/pnas.1219181110 [36] Liu, W.B., et al. (2013) Mitochondrial metabolism transi- tion cooperates with nuclear reprogramming during in- duced pluripotent stem cell generation. Biochemical and Biophysical Research Communications, 431, 767-771. doi:10.1016/j.bbrc.2012.12.148 [37] Mali, P., et al. (2010) Butyrate greatly enhances deriva- tion of human induced pluripotent stem cells by promot- ing epigenetic remodeling and the expression of pluripo- tency-associated genes. Stem Cells, 28, 713-720. [38] O nd e r, T. T., et al. (2012) Chromatin-modifying enzymes as modulators of reprogramming. Nature, 483, 598-602. [39] Lin, T.X., et al. (2009) A chemical platform for improved induction of human iPSCs. Nature Methods, 6, 805-808. [40] Valamehr, B., et al. (2012) A novel platform to enable the high-throughput derivation and characterization of fee- der-free human iPSCs. Scientific Reports, 2, 213. doi:10.1038/srep00213 [41] Wang, T., et al. (2011) The histone demethylases Jhdm1a/1b enhance somatic cell reprogramming in a vi- tamin-C-dependent manner. Cell Stem Cell, 9, 575-587. doi:10.1016/j.stem.2011.10.005 [42] Wang, Q., et al. (2011) Lithium, an anti-psychotic drug, greatly enhances the generation of induced pluripotent stem cells. Cell Research, 21, 1424-1435. doi:10.1038/cr.2011.108 [43] Zhu, S., Wei, W. and Ding, S. (2011) Chemical strategies for stem cell biology and regenerative medicine. Annual Review of Biomedical Engineering, 13, 73-90. doi:10.1146/annurev-bioeng-071910-124715 Copyright © 2013 SciRes. OPEN A CCES S
 P. E. Woolwine / Open Journal of Regenerati v e Medicine 2 (2013) 61- 73 71 [44] Lister, R., et al. (2011) Hotspots of aberrant epigenomic reprogramming in human induced pluripotent stem cells. Nature, 471, 68-73. [45] Ruiz, S., et al. (2012) Identification of a specific repro- gramming-associated epigenetic signature in human in- duced pluripotent stem cells. Proceedings of the National Academy of Sciences of the United States of America, 109, 16196-16201. doi:10.1073/pnas.1202352109 [46] Suhr, S.T., et al. (2009) Telomere dynamics in human cells reprogrammed to pluripotency. PLoS One, 4, e8124. doi:10.1371/journal.pone.0008124 [47] Prigione, A., Fauler, B., Lurz, R., Lehrach, H. and Adjaye, J. (2010) The senescence-related mitochondrial/oxidative stress pathway is repressed in human induced pluripotent stem cells. Stem Cells, 28, 721-733. doi:10.1002/stem.404 [48] Suhr, S.T., et al. (2010) Mitochondrial rejuvenation after induced pluripotency. PLoS One, 5, e14095. doi:10.1371/journal.pone.0014095 [49] Yoneyama, M., et al. (2004) The RNA helicase RIG-I has an essential function in double-stranded RNA-induced in- nate antiviral responses. Nature Immunology, 5, 730-737. [50] Angel, M. and Yanik, M.F. (2010) Innate immune sup- pression enables frequent transfection with RNA encod- ing reprogramming proteins. PLoS One, 5, e11756. doi:10.1371/journal.pone.0011756 [51] Warren, L., Ni, Y., Wang, J. and Guo, X. (2012) Feeder- free derivation of human induced pluripotent stem cells with messenger RNA. Scientific Reports, 2, 657. doi:10.1038/srep00657 [52] Their, M., Munst, B. and Edenhofer, F. (2010) Exploring refined conditions for reprogramming cells by recombi- nant Oct4 protein. The International Journal of Devel- opmental Biology, 54, 1713-1721. doi:10.1387/ijdb.103193mt [53] Their, M., Munst, B., Mielke, S. and Edenhofer, F. (2012) Cellular reprogramming employing recombinant Sox2 protein. Stem Cells International, 2012, Article ID: 549846. doi:10.1155/2012/549846 [54] Hook, L., et al. (2011) Non-immortalized human neural stem (NS) cells as a scalable platform for cellular assays. Neurochemistry International, 59, 432-444. doi:10.1016/j.neuint.2011.06.024 [55] Singh, A., et al. (2013) Adhesion strength-based, label- free isolation of human pluripotent stem cells. Nature Methods, 10, 438-444. [56] Street, C.A. and Bryan, B.A. (2011) Rho kinase proteins: Pleiotropic modulators of cell survival and apoptosis. Anticancer Research, 31, 3645-3657. [57] Hong, H., et al. (2009) Suppression of induced pluripo- tent stem cell generation by the p53-p21 pathway. Na- ture, 460, 1132-1135. [58] Yoshida, Y., Takahashi, K., Okita, K., Ichisaka, T. and Ya manaka, S. (2009) Hypoxia enhances the generation of induced pluripotent stem cells. Cell Stem Cell, 5, 237-241. doi:10.1016/j.stem.2009.08.001 [59] Covello, K.L., et al. (2006) HIF-2alpha regulates Oct-4: Effects of hypoxia on stem cell function, embryonic de- velopment, and tumor growth. Genes & Development, 20, 557-570. doi:10.1101/gad.1399906 [60] Zhu, S.Y., et al. (2010) Reprogramming of human pri- mary somatic cells by OCT4 and chemical compounds. Cell Stem Cell, 7, 651-655. doi:10.1016/j.stem.2010.11.015 [61] Staerk, J., et al. (2011) Pan-src family kinase inhibitors replace Sox2 during the direct reprogramming of somatic cells. Angewandte Chemie International Edition, 50, 5734-5736. doi:10.1002/anie.201101042 [62] Lian, X., Selekman, J., Bao, X., Hsiao, C., Zhu, K. and Palecek, S.P. (2013) A small molecule inhibitor of src family kinases promotes simple epithelial differentiation of human pluripotent stem cells. PLoS One, 8, e60016. doi:10.1371/journal.pone.0060016 [63] Kim, J.B., et al. (2009) Direct reprogramming of human neural stem cells by OCT4. Nature, 461, 649-653. [64] Hezroni, H., Sailaja, B.S. and Meshorer, E. (2011) Pluri- potency-related, valproic acid (VPA)-induced genome- wide histone H3 Lysine 9 (H3K9) acetylation patterns in embryonic stem cells. The Journal of Biological Chemis- try, 286, 35977-35988. doi:10.1074/jbc.M111.266254 [65] Huangfu, D., et al. (2008) Induction of pluripotent stem cells from primary human fibroblasts with only Oct4 and Sox2. Nature Biotechnology, 26, 1269-1275. [66] Wang, W.-P., et al. (2012) The EP300, KDM5A, KDM6A and KDM6B chromatin regulators cooperate with KLF4 in the transcriptional activation of POU5F1. PLoS One, 7, e52556. doi:10.1371/journal.pone.0052556 [67] Shi, Y., Desponts, C., Do, J.T., Hahm, H.S., Schöler, H.R. and Ding, S. (2008) Induction of pluripotent stem cells from mouse embryonic fibroblasts by Oct4 and Klf4 with small-molecule compounds. Cell Stem Cell, 3, 568-574. doi:10.1016/j.stem.2008.10.004 [68] Epsztejn-Litman, S., et al. (2008) De novo DNA methyl- lation promoted by G9a prevents reprogramming of em- bryonically silenced genes. Nature Structural & Molecu- lar Biology, 15, 1176-1183. [69] Vedadi, M., et al. (2011) A chemical probe selectively inhibits G9a and GLP methyltransferase activity in cells. Nature Chemical Biology, 7, 566-574. [70] Chen, X.J., et al. (2012) G9a/GLP-dependent histone H3K9me2 patterning during human hematopoietic stem cell lineage commitment. Genes & Develoment, 26, 2499- 2511. doi:10.1101/gad.200329.112 [71] Brueckner, B., et al. (2005) Epigenetic reactivation of tumor suppressor genes by a novel small-molecule in- hibitor of human DNA methyltransferases. Cancer Re- search, 65, 6305-6311. doi:10.1158/0008-5472.CAN-04-2957 [72] Niwa, H., Miyazaki, J. and Smith, A.G. (2000) Quantita- tive expression of Oct-3/4 defines differentiation, dedif- ferentiation or self-renewal of ES cells. Nature Genetics, 24, 372-376. [73] You, J.S., Kelly, T.K., De Carvalho, D.D., Taberlay, P.C., Liang, G. and Jones, P.A. (2011) OCT4 establishes and maintains nucleosome-depleted regions that provide addi- tional layers of epigenetic regulation of its target genes. Proceedings of the National Academy of Sciences of the Copyright © 2013 SciRes. OPEN A CCES S
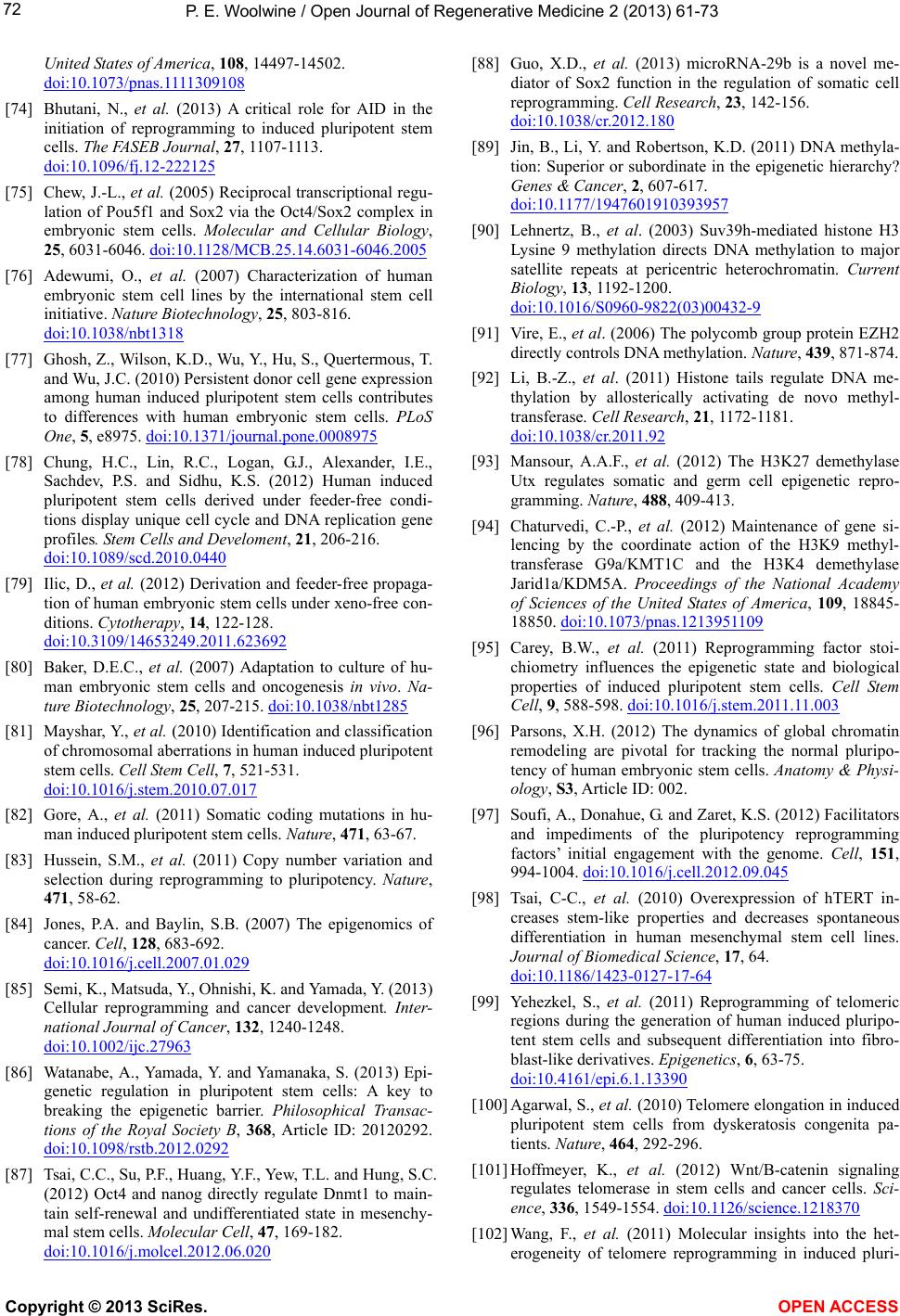 P. E. Woolwine / Open Journal of Regenerati v e Medicine 2 (2013) 61- 73 72 United States of America, 108, 14497-14502. doi:10.1073/pnas.1111309108 [74] Bhutani, N., et al. (2013) A critical role for AID in the initiation of reprogramming to induced pluripotent stem cells. The FASEB Journal, 27, 1107-1113. doi:10.1096/fj.12-222125 [75] Chew, J.-L., et al. (2005) Reciprocal transcriptional regu- lation of Pou5f1 and Sox2 via the Oct4/Sox2 complex in embryonic stem cells. Molecular and Cellular Biology, 25, 6031-6046. doi:10.1128/MCB.25.14.6031-6046.2005 [76] Adewumi, O., et al. (2007) Characterization of human embryonic stem cell lines by the international stem cell initiative. Nature Biotechnology, 25, 803-816. doi:10.1038/nbt1318 [77] Ghosh, Z., Wilson, K.D., Wu, Y., Hu, S., Quertermous, T. and Wu, J.C. (2010) Persistent donor cell gene expression among human induced pluripotent stem cells contributes to differences with human embryonic stem cells. PLoS One, 5, e8975. doi:10.1371/journal.pone.0008975 [78] Chung, H.C., Lin, R.C., Logan, G.J., Alexander, I.E., Sachdev, P.S. and Sidhu, K.S. (2012) Human induced pluripotent stem cells derived under feeder-free condi- tions display unique cell cycle and DNA replication gene profiles. Stem Cells and Develoment, 21, 206-216. doi:10.1089/scd.2010.0440 [79] Ilic, D., et al. (2012) Derivation and feeder-free propaga- tion of human embryonic stem cells under xeno-free con- ditions. Cytotherapy, 14, 122-128. doi:10.3109/14653249.2011.623692 [80] Baker, D.E.C., et al. (2007) Adaptation to culture of hu- man embryonic stem cells and oncogenesis in vivo. Na- ture Biotechnology, 25, 207-215. doi:10.1038/nbt1285 [81] Mayshar, Y., et al. (2010) Identification and classification of chromosomal aberrations in human induced pluripotent stem cells. Cell Stem Cell, 7, 521-531. doi:10.1016/j.stem.2010.07.017 [82] Gore, A., et al. (2011) Somatic coding mutations in hu- man induced pluripotent stem cells. Nature, 471, 63-67. [83] Hussein, S.M., et al. (2011) Copy number variation and selection during reprogramming to pluripotency. Nature, 471, 58-62. [84] Jones, P.A. and Baylin, S.B. (2007) The epigenomics of cancer. Cell, 128, 683-692. doi:10.1016/j.cell.2007.01.029 [85] Semi, K. , Matsuda, Y. , Oh n ishi, K. an d Yamada, Y. (2013) Cellular reprogramming and cancer development. Inter- national Journal of Cancer, 132, 1240-1248. doi:10.1002/ijc.27963 [86] Watanabe, A., Yamada, Y. and Yamanaka, S. (2013) Epi- genetic regulation in pluripotent stem cells: A key to breaking the epigenetic barrier. Philosophical Transac- tions of the Royal Society B, 368, Article ID: 20120292. doi:10.1098/rstb.2012.0292 [87] Tsai, C.C., Su, P.F., Huang, Y.F., Yew, T.L. and Hung, S.C. (2012) Oct4 and nanog directly regulate Dnmt1 to main- tain self-renewal and undifferentiated state in mesenchy- mal stem cells. Molecular Cell, 47, 169-182. doi:10.1016/j.molcel.2012.06.020 [88] Guo, X.D., et al. (2013) microRNA-29b is a novel me- diator of Sox2 function in the regulation of somatic cell reprogramming. Cell Research, 23, 142-156. doi:10.1038/cr.2012.180 [89] Jin, B., Li, Y. and Robertson, K.D. (2011) DNA methyla- tion: Superior or subordinate in the epigenetic hierarchy? Genes & Cancer, 2, 607-617. doi:10.1177/1947601910393957 [90] Lehnertz, B., et al. (2003) Suv39h-mediated histone H3 Lysine 9 methylation directs DNA methylation to major satellite repeats at pericentric heterochromatin. Current Biology, 13, 1192-1200. doi:10.1016/S0960-9822(03)00432-9 [91] Vire, E., et al. (2006) The polycomb group protein EZH2 directly controls DN A methylation. Nature, 439, 871-874. [92] Li, B.-Z., et al. (2011) Histone tails regulate DNA me- thylation by allosterically activating de novo methyl- transferase. Cell Research, 21, 1172-1181. doi:10.1038/cr.2011.92 [93] Mansour, A.A.F., et al. (2012) The H3K27 demethylase Utx regulates somatic and germ cell epigenetic repro- gramming. Nature, 488, 409-413. [94] Chaturvedi, C.-P., et al. (2012) Maintenance of gene si- lencing by the coordinate action of the H3K9 methyl- transferase G9a/KMT1C and the H3K4 demethylase Jarid1a/KDM5A. Proceedings of the National Academy of Sciences of the United States of America, 109, 18845- 18850. doi:10.1073/pnas.1213951109 [95] Carey, B.W., et al. (2011) Reprogramming factor stoi- chiometry influences the epigenetic state and biological properties of induced pluripotent stem cells. Cell Stem Cell, 9, 588-598. doi:10.1016/j.stem.2011.11.003 [96] Parsons, X.H. (2012) The dynamics of global chromatin remodeling are pivotal for tracking the normal pluripo- tency of human embryonic stem cells. Anatomy & Physi- ology, S3, Article ID: 002. [97] Soufi, A., Donahue, G. and Zaret, K.S. (2012) Facilitators and impediments of the pluripotency reprogramming factors’ initial engagement with the genome. Cell, 151, 994-1004. doi:10.1016/j.cell.2012.09.045 [98] Tsai, C-C., et al. (2010) Overexpression of hTERT in- creases stem-like properties and decreases spontaneous differentiation in human mesenchymal stem cell lines. Journal of Biomedical Science, 17, 64. doi:10.1186/1423-0127-17-64 [99] Yehezkel, S., et al. (2011) Reprogramming of telomeric regions during the generation of human induced pluripo- tent stem cells and subsequent differentiation into fibro- blast-like derivatives. Epigenetics, 6, 63-75. doi:10.4161/epi.6.1.13390 [100] Agarwal, S., et al. (2010) Telomere elongation in induced pluripotent stem cells from dyskeratosis congenita pa- tients. Nature, 464, 292-296. [101] Hoffmeyer, K., et al. (2012) Wnt/B-catenin signaling regulates telomerase in stem cells and cancer cells. Sci- ence, 336, 1549-1554. doi:10.1126/science.1218370 [102] Wang, F., et al. (2011) Molecular insights into the het- erogeneity of telomere reprogramming in induced pluri- Copyright © 2013 SciRes. OPEN A CCES S
 P. E. Woolwine / Open Journal of Regenerati v e Medicine 2 (2013) 61- 73 Copyright © 2013 SciRes. OPEN ACCESS 73 potent stem cells. Cell Research, 22, 757-768. doi:10.1038/cr.2011.201 [103] O’Callaghan, N.J. and Fenech, M. (2011) A quantitative PCR method for measuring absolute telomere length. Biological Procedures Online, 13, 3. doi:10.1186/1480-9222-13-3
|