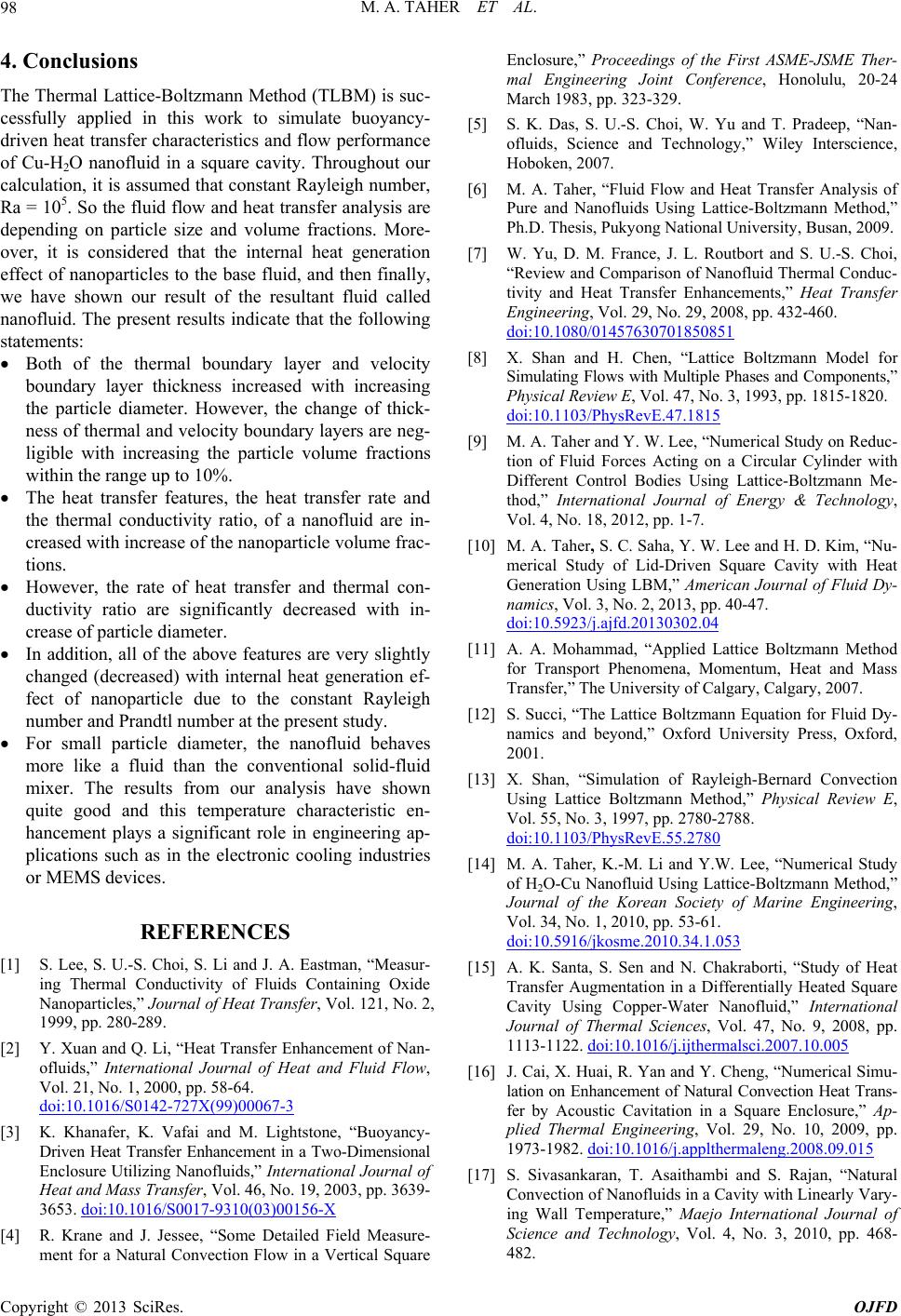
M. A. TAHER ET AL.
98
4. Conclusions
The Thermal Lattice-Boltzmann Method (TLBM) is suc-
cessfully applied in this work to simulate buoyancy-
driven heat transfer characteristics and flow performance
of Cu-H2O nanoflui
calculation, it is ass
d in a square cavity. Throughout our
umed that constant Rayleigh number,
ith increasing the particle volume fractions
ansfer and thermal con-
d (decreased) with internal heat generation
r, the nanofluid behaves
nal of Heat Transfer, Vol. 121, No. 2,
1999, pp. 280-289.
[2] Y. Xuan and Qhancement of Nan-
Ra = 105. So the fluid flow and heat transfer analysis are
depending on particle size and volume fractions. More-
over, it is considered that the internal heat generation
effect of nanoparticles to the base fluid, and then finally,
we have shown our result of the resultant fluid called
nanofluid. The present results indicate that the following
statements:
Both of the thermal boundary layer and velocity
boundary layer thickness increased with increasing
the particle diameter. However, the change of thick-
ness of thermal and velocity boundary layers are neg-
ligible w
within the range up to 10%.
The heat transfer features, the heat transfer rate and
the thermal conductivity ratio, of a nanofluid are in-
creased with increase of the nanoparticle volume frac-
tions.
However, the rate of heat tr
ductivity ratio are significantly decreased with in-
crease of particle diameter.
In addition, all of the above features are very slightly
change ef-
fect of nanoparticle due to the constant Rayleigh
number and Prandtl number at the present study.
For small particle diamete
more like a fluid than the conventional solid-fluid
mixer. The results from our analysis have shown
quite good and this temperature characteristic en-
hancement plays a significant role in engineering ap-
plications such as in the electronic cooling industries
or MEMS devices.
REFERENCES
[1] S. Lee, S. U.-S. Choi, S. Li and J. A. Eastman, “Measur-
ing Thermal Conductivity of Fluids Containing Oxide
Nanoparticles,” Jour
. Li, “Heat Transfer En
ofluids,” International Journal of Heat and Fluid Flow,
Vol. 21, No. 1, 2000, pp. 58-64.
doi:10.1016/S0142-727X(99)00067-3
[3] K. Khanafer, K. Vafai and M. Lightstone, “Buoyancy-
Driven Heat Transfer Enhancement in a Two-Dimensional
Enclosure Utilizing Nanofluids,” International Journal of
Heat and Mass Transfer, Vol. 46, No. 19, 2003, pp. 3639-
3653. doi:10.1016/S0017-9310(03)00156-X
deep, “Nan-
nal University, Busan, 2009.
ransfer Enhancements,” Heat Transfer
[4] R. Krane and J. Jessee, “Some Detailed Field Measure-
ment for a Natural Convection Flow in a Vertical Square
Enclosure,” Proceedings of the First ASME-JSME Ther-
mal Engineering Joint Conference, Honolulu, 20-24
March 1983, pp. 323-329.
[5] S. K. Das, S. U.-S. Choi, W. Yu and T. Pra
ofluids, Science and Technology,” Wiley Interscience,
Hoboken, 2007.
[6] M. A. Taher, “Fluid Flow and Heat Transfer Analysis of
Pure and Nanofluids Using Lattice-Boltzmann Method,”
Ph.D. Thesis, Pukyong Natio
[7] W. Yu, D. M. France, J. L. Routbort and S. U.-S. Choi,
“Review and Comparison of Nanofluid Thermal Conduc-
tivity and Heat T
Engineering, Vol. 29, No. 29, 2008, pp. 432-460.
doi:10.1080/01457630701850851
[8] X. Shan and H. Chen, “Lattice Boltzmann Model for
Simulating Flows with Multiple Phases and Components,”
Physical Review E, Vol. 47, No. 3, 1993, pp. 1815-1820.
doi:10.1103/PhysRevE.47.1815
[9] M. A. Taher and Y. W. Lee, “Numerical Study on
tion of Fluid Forces Acting on a C
Reduc-
ircular Cylinder with
ee and H. D. Kim, “Nu-
Different Control Bodies Using Lattice-Boltzmann Me-
thod,” International Journal of Energy & Technology,
Vol. 4, No. 18, 2012, pp. 1-7.
[10] M. A. Taher, S. C. Saha, Y. W. L
merical Study of Lid-Driven Square Cavity with Heat
Generation Using LBM,” American Journal of Fluid Dy-
namics, Vol. 3, No. 2, 2013, pp. 40-47.
doi:10.5923/j.ajfd.20130302.04
[11] A. A. Mohammad, “Applied Lattice Boltzmann Method
Press, Oxford,
for Transport Phenomena, Momentum, Heat and Mass
Transfer,” The University of Calgary, Calgary, 2007.
[12] S. Succi, “The Lattice Boltzmann Equation for Fluid Dy-
namics and beyond,” Oxford University
2001.
[13] X. Shan, “Simulation of Rayleigh-Bernard Convection
Using Lattice Boltzmann Method,” Physical Review E,
Vol. 55, No. 3, 1997, pp. 2780-2788.
doi:10.1103/PhysRevE.55.2780
[14] M. A. Taher, K.-M. Li and Y.W. Lee, “Numerical Study
of H2O-Cu Nanofluid Using Lattice-Boltzmann Method,”
Journal of the Korean Society of Marine Engineering,
Vol. 34, No. 1, 2010, pp. 53-61.
doi:10.5916/jkosme.2010.34.1.053
[15] A. K. Santa, S. Sen and N. Chakraborti, “Study of Heat
lsci.2007.10.005
Transfer Augmentation in a Differentially Heated Square
Cavity Using Copper-Water Nanofluid,” International
Journal of Thermal Sciences, Vol. 47, No. 9, 2008, pp.
1113-1122. doi:10.1016/j.ijtherma
ng, “Numerical Simu- [16] J. Cai, X. Huai, R. Yan and Y. Che
lation on Enhancement of Natural Convection Heat Trans-
fer by Acoustic Cavitation in a Square Enclosure,” Ap-
plied Thermal Engineering, Vol. 29, No. 10, 2009, pp.
1973-1982. doi:10.1016/j.applthermaleng.2008.09.015
[17] S. Sivasankaran, T. Asaithambi and S. Rajan, “Natural
Convection of Nanofluids in a Cavity with Linearly Vary-
ing Wall Temperature,” Maejo International Journal of
Science and Technology, Vol. 4, No. 3, 2010, pp. 468-
482.
Copyright © 2013 SciRes. OJFD