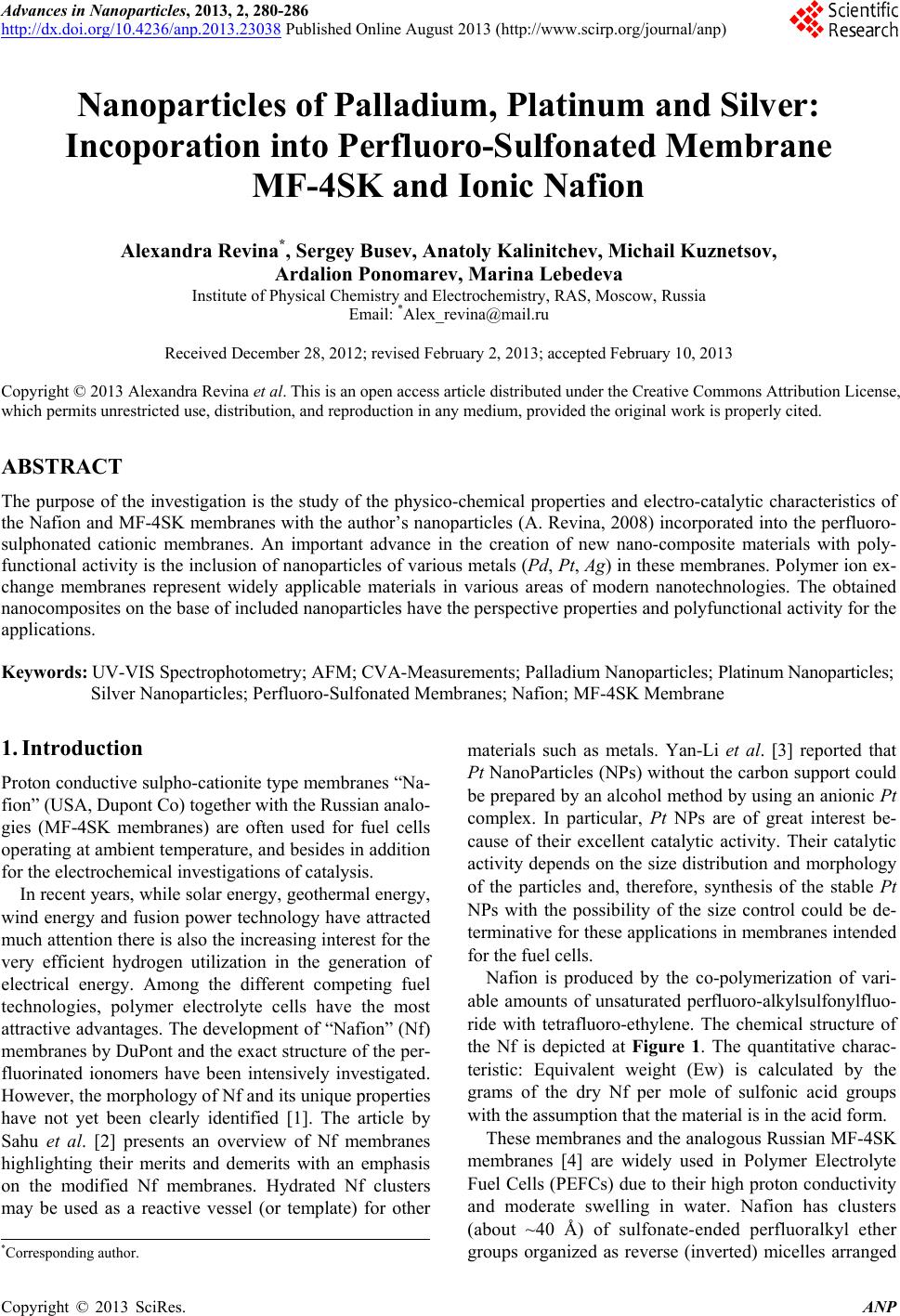
Advances in Nanoparticles, 2013, 2, 280-286
http://dx.doi.org/10.4236/anp.2013.23038 Published Online August 2013 (http://www.scirp.org/journal/anp)
Nanoparticles of Palladium, Platinum and Silver:
Incoporation into Perfluoro-Sulfonated Membrane
MF-4SK and Ionic Nafion
Alexandra Revina*, Sergey Busev, Anatoly Kalinitchev, Michail Kuznetsov,
Ardalion Ponomarev, Marina Lebedeva
Institute of Physical Chemistry and Electrochemistry, RAS, Moscow, Russia
Email: *Alex_revina@mail.ru
Received December 28, 2012; revised February 2, 2013; accepted February 10, 2013
Copyright © 2013 Alexandra Revina et al. This is an open access article distributed under the Creative Commons Attribution License,
which permits unrestricted use, distribution, and reproduction in any medium, provided the original work is properly cited.
ABSTRACT
The purpose of the investigation is the study of the physico-chemical properties and electro-catalytic characteristics of
the Nafion and MF-4SK membranes with the author’s nanoparticles (A. Revina, 2008) incorporated into the perfluoro-
sulphonated cationic membranes. An important advance in the creation of new nano-composite materials with poly-
functional activity is the inclusion of nanoparticles of various metals (Pd, Pt, Ag) in these membranes. Polymer ion ex-
change membranes represent widely applicable materials in various areas of modern nanotechnologies. The obtained
nanocomposites on the base of included nanoparticles have the perspective properties and polyfunctional activity for the
applications.
Keywords: UV-VIS Spectrophotometry; AFM; CVA-Measurements; Palladium Nanoparticles; Platinum Nanoparticles;
Silver Nanoparticles; Perfluoro-Sulfonated Membranes; Nafion; MF-4SK Membrane
1. Introduction
Proton conductive sulpho-cationite type membranes “Na-
fion” (USA, Dupont Co) together with the Russian analo-
gies (MF-4SK membranes) are often used for fuel cells
operating at ambient temperature, and besides in addition
for the electrochemical investigations of catalysis.
In recent years, while solar energy, geothermal energy,
wind energy and fusion power technology have attracted
much attention there is also the increasing interest for the
very efficient hydrogen utilization in the generation of
electrical energy. Among the different competing fuel
technologies, polymer electrolyte cells have the most
attractive advantages. The development of “Nafion” (Nf)
membranes by DuPont and the exact structure of the per-
fluorinated ionomers have been intensively investigated.
However, the morphology of Nf and its unique properties
have not yet been clearly identified [1]. The article by
Sahu et al. [2] presents an overview of Nf membranes
highlighting their merits and demerits with an emphasis
on the modified Nf membranes. Hydrated Nf clusters
may be used as a reactive vessel (or template) for other
materials such as metals. Yan-Li et al. [3] reported that
Pt NanoParticles (NPs) without the carbon support could
be prepared by an alcohol method by using an anionic Pt
complex. In particular, Pt NPs are of great interest be-
cause of their excellent catalytic activity. Their catalytic
activity depends on the size distribution and morphology
of the particles and, therefore, synthesis of the stable Pt
NPs with the possibility of the size control could be de-
terminative for these applications in membranes intended
for the fuel cells.
Nafion is produced by the co-polymerization of vari-
able amounts of unsaturated perfluoro-alkylsulfonylfluo-
ride with tetrafluoro-ethylene. The chemical structure of
the Nf is depicted at Figure 1. The quantitative charac-
teristic: Equivalent weight (Ew) is calculated by the
grams of the dry Nf per mole of sulfonic acid groups
with the assumption that the material is in the acid form.
These membranes and the analogous Russian MF-4SK
membranes [4] are widely used in Polymer Electrolyte
Fuel Cells (PEFCs) due to their high proton conductivity
and moderate swelling in water. Nafion has clusters
(about ~40 Å) of sulfonate-ended perfluoralkyl ether
groups organized as reverse (inverted) micelles arranged
*Corresponding author.
C
opyright © 2013 SciRes. ANP