 Journal of Behavioral and Brain Science, 2013, 3, 85-99 http://dx.doi.org/10.4236/jbbs.2013.31009 Published Online February 2013 (http://www.scirp.org/journal/jbbs) Neurodevelopmental Timing of Ethanol Exposure May Contribute to Observed Hetero geneity of Behavioral Deficits in a Mouse Model of Fetal Alcohol Spectrum Disorder (FASD) Katarzyna Mantha, Morgan Kleiber, Shiva Singh* Department of Biology, Molecular Genetics Unit, Western University, London, Canada Email: kjanus@uwo.ca, mlkleibe@uwo.ca, *ssingh@uwo.ca Received November 8, 2012; revised December 10, 2012; accepted December 17, 2012 ABSTRACT Maternal drinking during pregnancy can result in a wide spectrum of cognitive and behavioral abnormalities termed fetal alcohol spectrum disorders (FASD). The heterogeneity observed in FASD-related phenotypes can be attributed to a number of environmental and genetic factors; however, ethanol dose and timing of exposure may have significant influences. Here, we report the behavioral effects of acute, binge-like ethanol exposure at three neurodevelopmental times corresponding to the first, second, and third trimester of human development in C57BL/6J mice. Results show that developmental ethanol exposure consistently delays the development of basic motor skill reflexes and coordination as well as impairs spatial learning and memory. Observed changes in activity and anxiety-related behaviors, however, appear to be dependent on timing of alcohol exposure. The variability in behaviors between different treatment models suggests that these may be useful in evaluating the mechanisms disrupted by ethanol at specific neurodevelopmental times. The results provide further evidence that, regardless of developmental stage, the developing brain is acutely sen- sitive to alcohol exposure. Keywords: Fetal Alcohol Spectrum Disorder (FASD); Ethanol; Behavior; Neurodevelopment; Mouse Model; C57BL/6J 1. Introduction Maternal alcohol consumption during pregnancy can result in morphological, behavioral and neurological ab- normalities, collectively termed Fetal Alcohol Spectrum Disorders (FASD) [1,2]. The prevalence of FASD is es- timated to be approximately 1 in 100 live births in North America, and the occurrence and severity of FASD phe- notypes, including variable behavioral effects, have been attributed to the timing and dosage of alcohol [3-6]. The nature of the heterogeneity associated with variability of manifestation is poorly understood, as is the mechanism that causes these phenotypes to persist throughout the lifetime of an individual. These phenotypes often include delayed early-life development of motor control and co- ordination, hyperactivity, increased risk for anxiety-re- lated psychopathologies, impulsivity, inattentiveness and intellectual impairment [7-13]. Further, many children with FASD show deficits in cognition and learning, ex- ecutive functioning, memory and social adaptation [13- 17]. A number of animal models have been used to explore the relationship between ethanol exposure and specific FASD-related phenotypes [11,18-21]. Specifically, the generation of well-established behavioral battery proto- cols has led to a better characterization of behaviors and cognition in animals that have been prenatally exposure to alcohols. In particular, the C57BL/6J mouse has been shown to be acutely sensitive to both the physiological and the behavioral effects of neurodevelopmental ethanol exposure. This strain of mouse has been successfully used to replicate a number of FASD-relevant phenotypes in- cluding impairments in cognitive function, activity levels, novel-environment anxiety, and depression-related beha- viors [22-27]. Such results are considered representative of humans for a variety of reasons, including the fact that rodent and human neurodevelopmental timelines are com- parable [28-30], which allows for experimentation on timing-of-exposure [31-33]. Most previous work, how- ever, differs substantially in experimental factors such as ethanol dosage, timing of exposure, time of testing, be- *Corresponding author. C opyright © 2013 SciRes. JBBS
 K. MANTHA ET AL. 86 havioral phenotype evaluated, and testing protocol, mak- ing comparisons across studies difficult. Thisstudy is no- vel as it assesses the effects of exposure at specific neu- rodevelopmental times using a consistent dosage regi- ment. The results will provide a realistic framework for future studies. Specifically, we have used the C57BL/6J (B6) mouse strain to model the behavioral effects of acute, binge-like alcohol exposure at three neurodevelopmental time po- ints corresponding to human trimesters one (T1), two (T2) and three (T3). There is evidence that binge alcohol ex- posures are prevalent in pregnant women of certain high- risk groups, and can lead to FASD-related clinical diag- noses in resulting children [34,35]. We evaluated the re- sulting offspring across a battery of behavioral tests rele- vant to FASD-associated phenotypes from early neonatal development to adulthood. The results offer a perspective on the range of neurological and cognitive functions af- fected by alcohol that is dependent on timing of expo- sure. 2. Materials and Methods 2.1. Animals and Breeding Male and female C57BL/6J mice were originally ob- tained from Jackson Laboratories (Bar Harbor, ME, USE) and subsequently bred at the Health Sciences Animal Care Facility at the University of Western Ontario. All procedures involving the use of mice met the ethical standards outlined by the Canadian Council on Animal Care and were approved by the Animal Use Subcommit- tee of the University. Mice were housed in standard same- sex colony cages with a temperature range of 21˚C - 24˚C and at 14-h light/10-h dark schedule, with free ac- cess to food and water. For breeding, females of appro- ximately eight weeks of age were housed in individual cages and time-mated overnight with 8 - 12 weeks old males. At the end of the mating period, females were examined for presence of a vaginal plug, indicating ges- tational day 0 (G0), and males were removed from cages. 2.2. Ethanol Injections Pregnant females were randomly assigned to two groups in each of the three trimesters: control dams injected subcutaneously (abdomen) with 0.15 M saline solution and ethanol-treated dams injected with 20% ethanol in the saline solution at the same site. An injection of 2.5 g/kg was given twice, spaced two hours apart to model a heavy, binge-like exposure (blood alcohol concentration or BAC remains over 200 mg/dl for at least 4 hours) [29]. Subcutaneous injections were given on G8 and G11 to model the first trimester [36,37], and on G14 and G16 to model the second trimester equivalent [31,38]. In order to model for the third trimester equivalent, pups at post- natal day 4 (P4) and P7 were subcutaneously injected us- ing the same dosage. This stage parallels the human equi- valent of third trimester alcohol exposure [28,29,39]. It is not possible to perfectly match such stages across species. Consequently, these timings should be viewed as the best approximation for the human equivalent. Dams were ran- domly assigned to control and ethanol-treated groups. Third trimester pups from each litter were matched where possible to control for litter effects. The number of litters used for T1 include 4 ethanol and 3 control litters, and 6 ethanol and 4 control litters for T2. For T3 we were able to assign pups from the same litter to two treatments from a total of 6 litters. Dams and pups were monitored following ethanol injections until full recovery, and pups were weaned on P21 into colony cages of two to four same-sex littermates. All pups were run through the same battery of tests and treated as statistically independent observations. 2.3. Early Postnatal Development From P2 to P21, pups were assessed for the ability to reach critical developmental milestones evaluating the appearance of age-appropriate motor skills such as bal- ance, motor coordination, strength and reflexes, follow- ing Hill and colleagues [40]. Tests were performed at the same time each day until the pup was able to perform the task in the prescribed amount of time for two consecutive days [41]. The tests used included surface righting, nega- tive geotaxis, cliff aversion, forelimb grasp, auditory star- tle, ear twitch, open field traversal, air righting and eye opening. Pups from T3 (P4 and P7) underwent develop- mental milestone testing beginning at 9:30 am, followed by injections at 12:00 pm. 2.4. Open-Field Locomotor Activity to Test Activity and Anxiety At P25 pups were assessed for activity in a novel open- field environment using the infrared Actimeter system and measured using Acti-Track software (Panlab, Barce- lona, Spain) [41]. We chose mice at young adolescence following previous reports that this testing during this period results in measurable differences between etha- nol-treated and control mice [41]. Also, this timing cor- responds to the prepubescent period of development in mice [42]. The prepubescent period is the time markedly used in human studies assessing disorders involving hy- peractive behaviors [43,44]. The open field arena con- sisted of a 45 cm (W) × 45 cm (L) surface constructed of black plexiglass enclosed by four 35 cm-high clear acry- lic walls, as well as an infrared frame that produced a 16 × 16 grid of intersecting beams used to track the move- ment of each mouse. Infrared beam-break data were used Copyright © 2013 SciRes. JBBS
 K. MANTHA ET AL. 87 to calculate locomotor activity. Movement data were also analyzed by dividing the arena into an outer periphery zone and a central zone to allow for the evaluation of thigmotaxis. Testing was conducted during the light phase between 1000 h and 1300 h, and the lighting of the arena was 100 lx. Each mouse was placed in the same corner of the arena when beginning the trial, and was allowed to freely explore for 15 min. At the end of the testing, the mouse was removed and returned to its home cage. Be- tween trials, the arena was cleaned with 30% isopropa- nol. 2.5. Home Cage Activity Testing Activity in a familiar environment was measured using the infrared Actimeter system (Panlab, Barcelona, Spain). At P35, mice were placed individually into 38 cm (L) × 24 cm (W) × 14 cm (H) transparent plastic cages (Inno- vive, San Diego, CA, USA) with standard woodchip bed- ding and free access to food and water. Following a 24 h acclimation period, cages were placed in the Actimeter frame and testing was conducted overnight from 1900 h to 0600 h, spanning the dark phase of the light/dark cycle and 1 h of light at the beginning. Recordings were taken for two consecutive nights and averaged. Mice were re- housed in their original cages at the end of activity test- ing. 2.6. Light/Dark Box for Testing Anxiety The light/dark box was used as a measure of exploratory behavior and anxiety in a novel, illuminated environment to further characterize the thigmotaxis behavior observed during the open-field test. The apparatus was constructed following Crawley and colleagues [45] and included two compartments consisting of a 27 cm (L) × 27 cm (W) × 27 cm (H) light arena and a 18 cm (L) × 27 cm (W) × 27 cm (H) dark arena, with a 7.5 cm × 7.5 cm opening be- tween the light and dark regions. At age P40, each mouse was placed in the light arena facing the opening, and al- lowed to freely explore both light and dark areas for 5 min. The overhead light in the room was 200 lx and all trials were recorded by a ceiling-mounted camera. Any- Maze digital tracking software (Stoelting, Wood Dale, IL, USA) was used to analyze movements and track the amount of time spent in the light arena versus the dark arena. Following testing, the mouse was returned to its home cage. 2.7. Barnes Maze to Test Spatial Learning and Memory A modified version of Barnes maze for mice was con- structed following Sunyer and colleagues [46] and was conducted as previously described [41]. Briefly, the test consisted of four 3-minute training trials per day, spaced 15 minutes apart, over four consecutive days beginning at P50 (acquisition days). For each trial, the mouse was placed in a start chamber in the centre of the platform, and after 10 seconds, an overhead 220 lx light and 85 dB computer-generated white noise were turned on, and the mouse was released to explore the platform. The trial ended once the mouse successfully located the target and entered the escape box. The light and white noise were terminated immediately after entry. If the 3 minutes had elapsed without the mouse entering the escape box, the mouse was guided into the box by the experimenter and left for 1 minute undisturbed. Between trials, the plat- form was cleaned with 30% isopropanol. Trials were also qualitatively scored based on whether the mouse used a direct strategy (mouse moves directly to the escape hole or an adjacent hole), a serial strategy (the first visit to the escape box was preceded by visiting at least two adjacent holes in a serial manner, in a clockwise or counter-clock- wise direction), or a mixed strategy (hole explorations were separated by crossing through the centre of the maze or unorganized search). The day following the last acquisition day (day 5 of testing), the escape box was covered and the mouse was allowed to explore the maze for 1 minute before being returned to its home cage (probe trials). The number of errors and explorations to the target hole were recorded. The same probe trial protocol was also performed seven days following the first probe trial (day 12 of testing) without further training between these days. Trials were recorded using a ceiling-mounted camera and analyzed using AnyMaze digital tracking software (Stoelting, Wood Dale, IL, USA) for latency to reach the escape box. Ex- plorations and search strategy was scored manually by an independent observer unaware of the treatment group. 2.8. Statistical Analysis Data were analyzed using appropriate analysis of vari- ance methods depending of the number of independent variables (sex, treatment, repeated day of testing). Where data were analyzed across days, repeated-measures ana- lysis of variance (ANOVA) with treatment as the be- tween-subjects factor and day as the within-subjects fac- tor was used. For overnight activity, we performed hypo- thesis-based, step-down analyses and corrected for mul- tiple testing. All data are reported as mean ± standard error of the mean. For the Barnes maze acquisition days, data were log3-transformed to account for the differences in variance between latencies to reach the target across days. Data analysis was stratified by sex if sexually di- morphic effects were observed. Effects of litter were ana- lyzed using “litter” as a covariate in the ANOVA, how- ever, no litter effects were observed for any of the beha- Copyright © 2013 SciRes. JBBS
 K. MANTHA ET AL. 88 vioral measures. All statistical analyses were performed using SPSS v.16 (SPSS Inc, Chicago, IL, USA). 3. Results 3.1. Postnatal Developmental Milestones All pups were evaluated from P2 to P21 for the abilities to reach critical neurodevelopmental milestones. The ave- rage day each specific milestone was achieved by each treatment group is shown in Table 1. The ethanol expo- sure during T1 significantly delayed the ability of pups to surface right themselves when placed on their backs (F1,46 = 29.90, p < 0.001), grasp a rod with their fore- limbs (F1,46 = 15.50, p < 0.001), extinguish pivoting be- havior and transverse out of a 15 cm-diameter circle (F1,46 = 15.10, p < 0.001), and right themselves in the air when dropped from upside down from 5 cm (F1,46 = 23.71, p < 0.001). Interestingly, a significant interaction between sex and treatment was observed for the ability of the pup to right itself in the air when dropped (F1,46 = 7.60, p = 0.008), with control males taking longer to right themselves than control females but exposed females tak- ing longer to right themselves than exposed males. T2 ethanol-treated pups were significantly delayed in time it took to surface right (F1,54 = 4.93, p = 0.03), turn 180˚ upward when placed downward on a screen set at 45˚ (F1,54 = 34.88, p < 0.001), crawl away from the edge of a cliff (F1,54 = 25.10, p < 0.001), grasp the rod (F1,54 = 6.65, p = 0.01), transverse out of the circle (F1,54 = 17.02, p < 0.001), right themselves in the air (F1,54 = 10.06, p = 0.003) and open their eyes for the first time (F1,54 = 6.21, p = 0.02). Finally, T3 ethanol-treated pups had signifi- cant delays in surface righting (F1,39 = 97.15, p < 0.001), grasping the rod (F1,39 = 10.96, p = 0.002), reacting to a handclap at a distance of 10 cm (F1,39 = 9.52, p = 0.004), flattening their ear in response to an applicator (F1,39 = 6.34, p = 0.02), traversing outside of the circle (F1,39 = 27.67, p < 0.001), opening their eyes (F1,39 = 12.72, p < 0.001), and righting in the air (F1,39 = 63.24, p < 0.001). 3.2. Locomotor Activity and Anxiety-Related Traits Spontaneous activity of juvenile (P25) offspring from control and ethanol-treated mice for each trimester was assessed over a 15 minute period in a novel open-field environment. Two-way ANOVA did not result in a sig- nificant effect of sex or an interaction between sex and treatment, therefore the groups were collapsed and a one- way ANOVA with treatment as the main factor was ap- plied. It showed a significant effect of treatment in T1 (F1,46 = 6.48, p = 0.01) and T2 (F1,55 = 69.89, p < 0.001), where the number of beam breaks was increased in etha- nol-treated mice versus control mice (Figures 1(a) and (b)). No significant effect of treatment was observed for T3 mice (Figure 1(c)). To evaluate the effects of novelty induced anxiety-re- lated traits in the open field task, we also examined dif- ferences in thigmotaxis during open-field testing. Al- though no statistically significant effect of treatment was observed for T1 mice (Figure 2(a)), a significant main effect of treatment was observed between T2 exposed and control mice (F1,55 = 59.65, p < 0.001), with ethanol- treated offspring spending significantly more time in the centre zone than control mice (Figure 2(b)). Interest- ingly, mice treated with ethanol during the T3 equivalent spent significantly less time than control mice in the centre Figure 1. Locomotor activity in a novel open-field environ- ment. Mean (±SEM) infrared beam breaks of ethanol-trea- ted and control mice from trimester 1 (a), trimester 2 (b) and trimester 3 (c) over a 15 min period (n = 21 - 31 mice per group). *p < 0.05; ***p < 0.001. Copyright © 2013 SciRes. JBBS
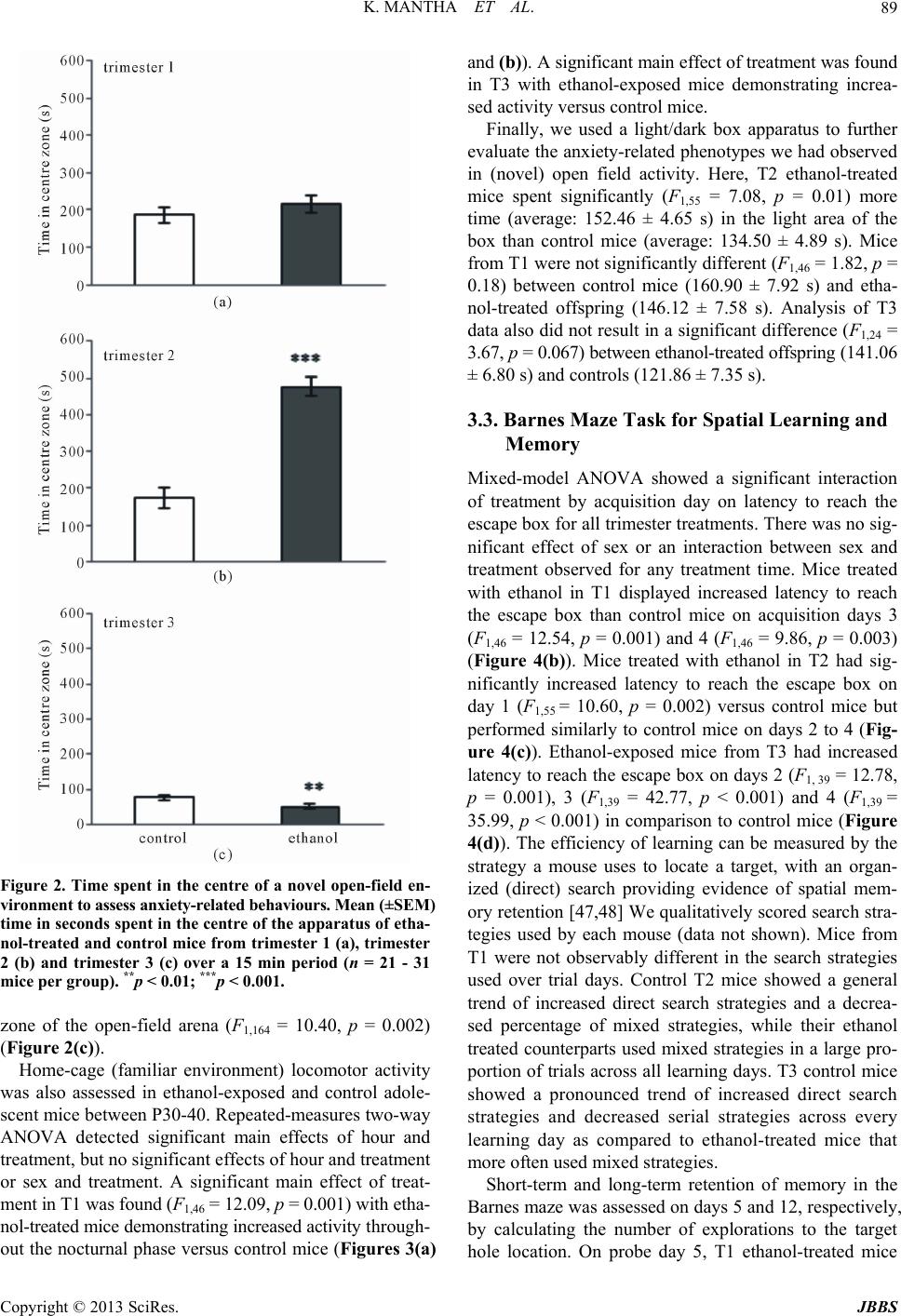 K. MANTHA ET AL. 89 Figure 2. Time spent in the centre of a novel open-field en- vironment to assess anxiety-related behaviours. Mean (±SEM ) time in seconds spent in the centre of the apparatus of etha- nol-treated and control mice from trimester 1 (a), trimester 2 (b) and trimester 3 (c) over a 15 min period (n = 21 - 31 mice per group). **p < 0.01; ***p < 0.001. zone of the open-field arena (F1,164 = 10.40, p = 0.002) (Figure 2(c)). Home-cage (familiar environment) locomotor activity was also assessed in ethanol-exposed and control adole- scent mice between P30-40. Repeated-measures two-way ANOVA detected significant main effects of hour and treatment, but no significant effects of hour and treatment or sex and treatment. A significant main effect of treat- ment in T1 was found (F1,46 = 12.09, p = 0.001) with etha- nol-treated mice demonstrating increased activity through- out the nocturnal phase versus control mice (Figures 3(a) and (b)). A significant main effect of treatment was found in T3 with ethanol-exposed mice demonstrating increa- sed activity versus control mice. Finally, we used a light/dark box apparatus to further evaluate the anxiety-related phenotypes we had observed in (novel) open field activity. Here, T2 ethanol-treated mice spent significantly (F1,55 = 7.08, p = 0.01) more time (average: 152.46 ± 4.65 s) in the light area of the box than control mice (average: 134.50 ± 4.89 s). Mice from T1 were not significantly different (F1,46 = 1.82, p = 0.18) between control mice (160.90 ± 7.92 s) and etha- nol-treated offspring (146.12 ± 7.58 s). Analysis of T3 data also did not result in a significant difference (F1,24 = 3.67, p = 0.067) between ethanol-treated offspring (141.06 ± 6.80 s) and controls (121.86 ± 7.35 s). 3.3. Barnes Maze Task for Spatial Learning and Memory Mixed-model ANOVA showed a significant interaction of treatment by acquisition day on latency to reach the escape box for all trimester treatments. There was no sig- nificant effect of sex or an interaction between sex and treatment observed for any treatment time. Mice treated with ethanol in T1 displayed increased latency to reach the escape box than control mice on acquisition days 3 (F1,46 = 12.54, p = 0.001) and 4 (F1,46 = 9.86, p = 0.003) (Figure 4(b)). Mice treated with ethanol in T2 had sig- nificantly increased latency to reach the escape box on day 1 (F1,55 = 10.60, p = 0.002) versus control mice but performed similarly to control mice on days 2 to 4 (Fig- ure 4(c)). Ethanol-exposed mice from T3 had increased latency to reach the escape box on days 2 (F1, 39 = 12.78, p = 0.001), 3 (F1,39 = 42.77, p < 0.001) and 4 (F1,39 = 35.99, p < 0.001) in comparison to control mice (Figure 4(d)). The efficiency of learning can be measured by the strategy a mouse uses to locate a target, with an organ- ized (direct) search providing evidence of spatial mem- ory retention [47,48] We qualitatively scored search stra- tegies used by each mouse (data not shown). Mice from T1 were not observably different in the search strategies used over trial days. Control T2 mice showed a general trend of increased direct search strategies and a decrea- sed percentage of mixed strategies, while their ethanol treated counterparts used mixed strategies in a large pro- portion of trials across all learning days. T3 control mice showed a pronounced trend of increased direct search strategies and decreased serial strategies across every learning day as compared to ethanol-treated mice that more often used mixed strategies. Short-term and long-term retention of memory in the Barnes maze was assessed on days 5 and 12, respectively, by calculating the number of explorations to the target hole location. On probe day 5, T1 ethanol-treated mice Copyright © 2013 SciRes. JBBS
 K. MANTHA ET AL. Copyright © 2013 SciRes. JBBS 90 Figure 3. Locomotor activity in a familiar home-cage environment stratified by sex. Mean (±SEM) infrared beam breaks by ethanol-exposed and control mice from trimester 1 males (a) and females (b), trimester 2 males (c) and females (d), and tri- mester 3 males (e) and females (f) over an 11-h period (n = 9 - 20 mice per group). *p < 0.05; **p < 0.01; ***p < 0.001. were not significantly different from controls in the num- ber of explorations to the escape box (Figure 4(a)). Ge- nerally, ethanol-treated mice displayed increased explo- rations to the opposite end of the target than control mice (Figures 5(a) and (b)). On probe day 12, we found a main effect of sex (F1,46 = 4.22, p = 0.046), with females exhibiting more explorations to the target (average fe- male: 2.64 ± 0.15 explorations) than males (average male: 2.21 ± 0.15 explorations). No significant effects of sex or an interaction between sex and treatment was observed for the explorations of ethanol-treated and control T2 mice on probe day 5. Sig- nificant effects of treatment were observed for the ex- plorations to the escape hole location (F1,55 = 5.21, p = 0.03) and hole position −1 (F1,55 = 14.27, p < 0.001), with control mice displaying increased exploration around the target than exposed mice (Figure 5(c)). Ethanol-treated ice exhibited more explorations on the opposite site of m
 K. MANTHA ET AL. 91 Figure 4. Latency to reach the target in the Barnes maze (a) used for spatial learning and memory. Average latencies to the target represent mean (±SEM) of four trials per day across four acquisition days for ethanol-treated and control mice from trimester 1 (b), trimester 2 (c) and trimester 3 (d). Data show n are c oll apse d acr oss se x ( n = 21 - 31 mice per group). **p < 0.01; ***p < 0.001. the target zone than control mice (Figure 5(c)). Signifi- cant effects of treatment were observed for the explora- tions to the escape hole (F1,55 = 12.29, p = 0.001), with control mice spending more time within the target area than ethanol-treated mice (Figure 5(d)). For T3 probe days 5 and 12 explorations, we did not observe any significant effects of sex or an interaction between sex and treatment. A significant effect of treat- ment was observed on probe day 5 for explorations to the target (F1,39 = 14.22, p < 0.001) and positions near the target, with control mice displaying significantly more explorations around the target than ethanol-treated mice (Figure 5(e)). We also found a significant effect of treat- ment on probe day 12 for the target hole (F1,39 = 7.80, p = 0.008), with control mice spending more time in the tar- get area than ethanol-treated mice Figure 5(f)). 4. Discussion This study evaluates the effects of heavy binge-like etha- nol exposure at neurodevelopmental times approximating human trimesters one, two and three across a battery of behavioral assays. The phenotypes assessed fall into three categories: motor skill development, locomotor activity including behaviors relevant to anxiety, and spatial learn- ing and memory. The specific assays are well-established and have been used extensively in the literature to model human behaviors that are most relevant to FASD [40,46, 49-53]. Our results show that ethanol exposure causes changes in a number of behaviors examined and that the extent of alterations may be dependent upon the gesta- tional timing of ethanol treatment. 4.1. Motor Skill Development The results show that ethanol exposure at any time dur- ing gestation may cause delays in motor skill and reflex development. Specifically, our results show delays in sur- face righting, open-field traversal and air righting (Table ), which follows the literature [54,55]. Such phenotypes 1 Copyright © 2013 SciRes. JBBS
 K. MANTHA ET AL. 92 Figure 5. Number of explorations for control and ethanol-treated mice during Barnes maze on days 5 and 12 to represent short-term and long-term memory retention, respectively. Mean (±SEM) number of explorations to each hole of the Barnes maze for ethanol-treated and control mic e on day 5 for trimester 1 (a), trimester 2 (c) and trimester 3 (e), and day 12 for tri- mester 1 (b), trimester 2 (d) and trimester 3 (f). The position of numbered holes (x-axis) of Barnes maze are shown in Figure 3(a). Data shown here are collapsed across sex (n = 21 - 31 mice per group). *p < 0.05; **p < 0.01; ***p < 0.001. are early indicators of the rostro-caudal gradient of limb coordination maturation [56]. Ethanol exposure during the trimester three equivalent appears to produce delays in most measures, followed closely by trimester two, while ethanol exposure at the first trimester produced subtle effects, with less than half of the milestones significantly altered. This pattern may suggest that the brain regions responsible for the development of these neuro-motor skills may be less sensitive to ethanol at early stages of neurodevelopment, such as neurulation and cell prolif- eration [32]. Also, fetal ethanol exposure can cause cell death and neuronal reduction [57-62], that is known to lead to motor skill deficits [63]. Further, the impairments caused by late gestation alcohol exposure may be attrib- uted to specific brain region development including syn- apse formation in the cerebellum and prefrontal cortex [33,61,63-65]. Such results are relevant to FASD as young children with FASD also show delayed motor de- velopment and fine-motor dysfunction including weak grasp, poor hand-eye coordination and poor balance [66- 70], which is thought to result from damage to the cere- ellum given its involvement in motor function control b Copyright © 2013 SciRes. JBBS
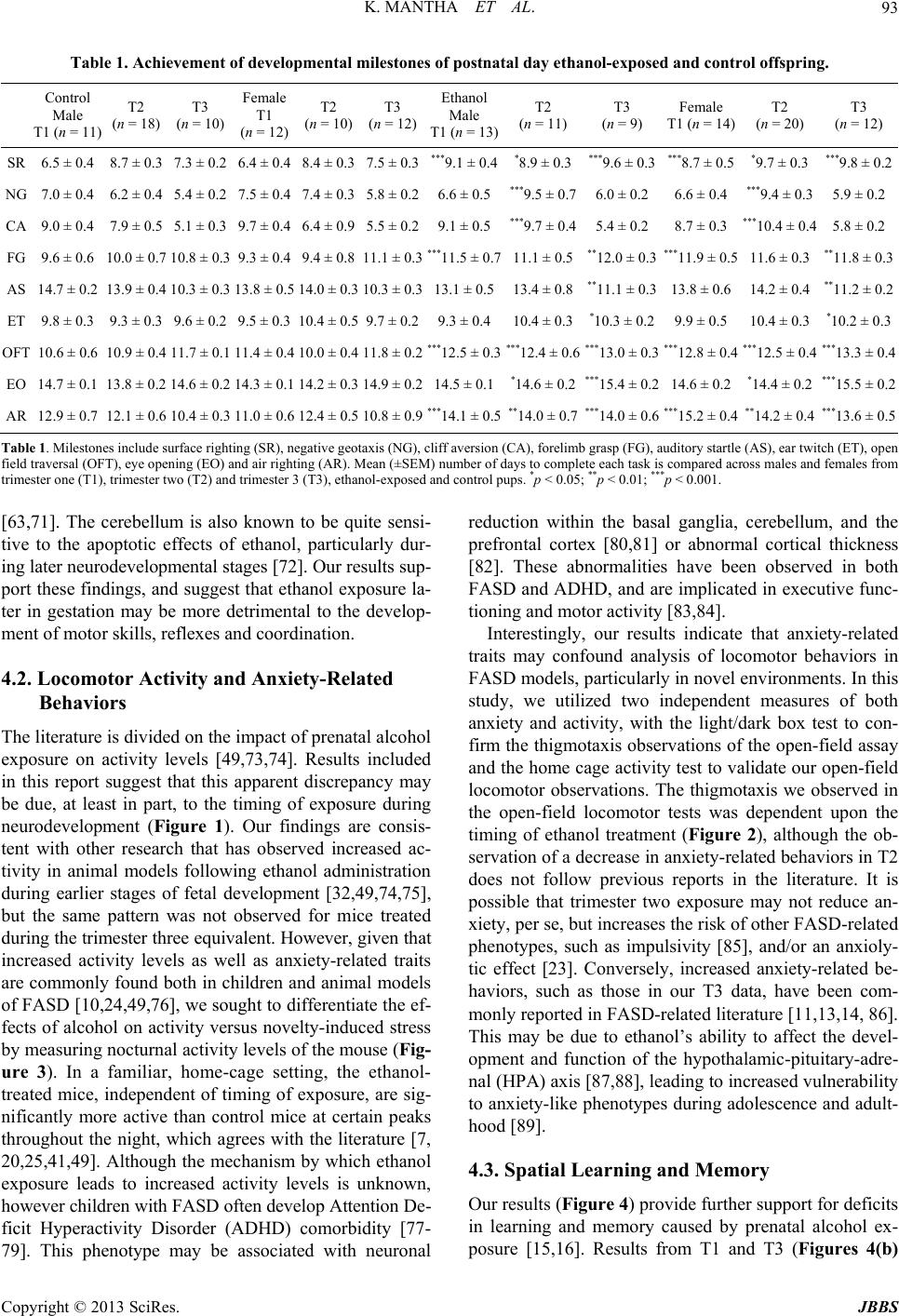 K. MANTHA ET AL. 93 Table 1. Achievement of developmental milestones of postnatal day ethanol-exposed and control offspring. Control Male T1 (n = 11) T2 (n = 18) T3 (n = 10) Female T1 (n = 12) T2 (n = 10) T3 (n = 12) Ethanol Male T1 (n = 13) T2 (n = 11) T3 (n = 9) Female T1 (n = 14) T2 (n = 20) T3 (n = 12) SR 6.5 ± 0.4 8.7 ± 0.3 7.3 ± 0.26.4 ± 0.4 8.4 ± 0.37.5 ± 0.3***9.1 ± 0.4*8.9 ± 0.3***9.6 ± 0.3***8.7 ± 0.5 *9.7 ± 0.3 ***9.8 ± 0.2 NG 7.0 ± 0.4 6.2 ± 0.4 5.4 ± 0.27.5 ± 0.4 7.4 ± 0.35.8 ± 0.26.6 ± 0.5***9.5 ± 0.76.0 ± 0.26.6 ± 0.4 ***9.4 ± 0.35.9 ± 0.2 CA 9.0 ± 0.4 7.9 ± 0.5 5.1 ± 0.39.7 ± 0.4 6.4 ± 0.95.5 ± 0.29.1 ± 0.5***9.7 ± 0.45.4 ± 0.28.7 ± 0.3 ***10.4 ± 0.45.8 ± 0.2 FG 9.6 ± 0.6 10.0 ± 0.7 10.8 ± 0.39.3 ± 0.4 9.4 ± 0.811.1 ± 0.3 ** *11.5 ± 0.711.1 ± 0.5**12.0 ± 0.3***11.9 ± 0.5 11.6 ± 0.3 **11.8 ± 0.3 AS 14.7 ± 0.2 13.9 ± 0.4 10.3 ± 0.3 13.8 ± 0.5 14.0 ± 0.3 10.3 ± 0.313.1 ± 0.513.4 ± 0.8**11.1 ± 0.313.8 ± 0.6 14.2 ± 0.4 **11.2 ± 0.2 ET 9.8 ± 0.3 9.3 ± 0.3 9.6 ± 0.29.5 ± 0.3 10.4 ± 0.59.7 ± 0.29.3 ± 0.410.4 ± 0.3*10.3 ± 0.29.9 ± 0.5 10.4 ± 0.3 *10.2 ± 0.3 OFT 10.6 ± 0.6 10.9 ± 0.4 11.7 ± 0.1 11.4 ± 0.4 10.0 ± 0.4 11.8 ± 0.2 ***12.5 ± 0.3** *12.4 ± 0.6** *13.0 ± 0.3***12.8 ± 0.4 ***12.5 ± 0.4***13.3 ± 0.4 EO 14.7 ± 0.1 13.8 ± 0.2 14.6 ± 0.2 14.3 ± 0.1 14.2 ± 0.3 14.9 ± 0.214.5 ± 0.1*14.6 ± 0.2***15.4 ± 0.214.6 ± 0.2 *14.4 ± 0.2***15.5 ± 0.2 AR 12.9 ± 0.7 12.1 ± 0.6 10.4 ± 0.3 11.0 ± 0.6 12.4 ± 0.5 10.8 ± 0.9 ***14.1 ± 0.5**14.0 ± 0.7***14.0 ± 0.6** *15.2 ± 0.4 **14.2 ± 0.4***13.6 ± 0.5 Table 1. Milestones include surface righting (SR), negative geotaxis (NG), cliff aversion (CA), forelimb grasp (FG), auditory startle (AS), ear twitch (ET), open field traversal (OFT), eye opening (EO) and air righting (AR). Mean (±SEM) number of days to complete each task is compared across males and females from trimester one (T1), trimester two (T2) and trimester 3 (T3), ethanol-exposed and control pups. *p < 0.05; **p < 0.01; ***p < 0.001. [63,71]. The cerebellum is also known to be quite sensi- tive to the apoptotic effects of ethanol, particularly dur- ing later neurodevelopmental stages [72]. Our results sup- port these findings, and suggest that ethanol exposure la- ter in gestation may be more detrimental to the develop- ment of motor skills, reflexes and coordination. 4.2. Locomotor Activity and Anxiety-Related Behaviors The literature is divided on the impact of prenatal alcohol exposure on activity levels [49,73,74]. Results included in this report suggest that this apparent discrepancy may be due, at least in part, to the timing of exposure during neurodevelopment (Figure 1). Our findings are consis- tent with other research that has observed increased ac- tivity in animal models following ethanol administration during earlier stages of fetal development [32,49,74,75], but the same pattern was not observed for mice treated during the trimester three equivalent. However, given that increased activity levels as well as anxiety-related traits are commonly found both in children and animal models of FASD [10,24,49,76], we sought to differentiate the ef- fects of alcohol on activity versus novelty-induced stress by measuring nocturnal activity levels of the mouse (Fig- ure 3). In a familiar, home-cage setting, the ethanol- treated mice, independent of timing of exposure, are sig- nificantly more active than control mice at certain peaks throughout the night, which agrees with the literature [7, 20,25,41,49]. Although the mechanism by which ethanol exposure leads to increased activity levels is unknown, however children with FASD often develop Attention De- ficit Hyperactivity Disorder (ADHD) comorbidity [77- 79]. This phenotype may be associated with neuronal reduction within the basal ganglia, cerebellum, and the prefrontal cortex [80,81] or abnormal cortical thickness [82]. These abnormalities have been observed in both FASD and ADHD, and are implicated in executive func- tioning and motor activity [83,84]. Interestingly, our results indicate that anxiety-related traits may confound analysis of locomotor behaviors in FASD models, particularly in novel environments. In this study, we utilized two independent measures of both anxiety and activity, with the light/dark box test to con- firm the thigmotaxis observations of the open-field assay and the home cage activity test to validate our open-field locomotor observations. The thigmotaxis we observed in the open-field locomotor tests was dependent upon the timing of ethanol treatment (Figure 2), although the ob- servation of a decrease in anxiety-related behaviors in T2 does not follow previous reports in the literature. It is possible that trimester two exposure may not reduce an- xiety, per se, but increases the risk of other FASD-related phenotypes, such as impulsivity [85], and/or an anxioly- tic effect [23]. Conversely, increased anxiety-related be- haviors, such as those in our T3 data, have been com- monly reported in FASD-related literature [11,13,14, 86]. This may be due to ethanol’s ability to affect the devel- opment and function of the hypothalamic-pituitary-adre- nal (HPA) axis [87,88], leading to increased vulnerability to anxiety-like phenotypes during adolescence and adult- hood [89]. 4.3. Spatial Learning and Memory Our results (Figure 4) provide further support for deficits in learning and memory caused by prenatal alcohol ex- posure [15,16]. Results from T1 and T3 (Figures 4(b) Copyright © 2013 SciRes. JBBS
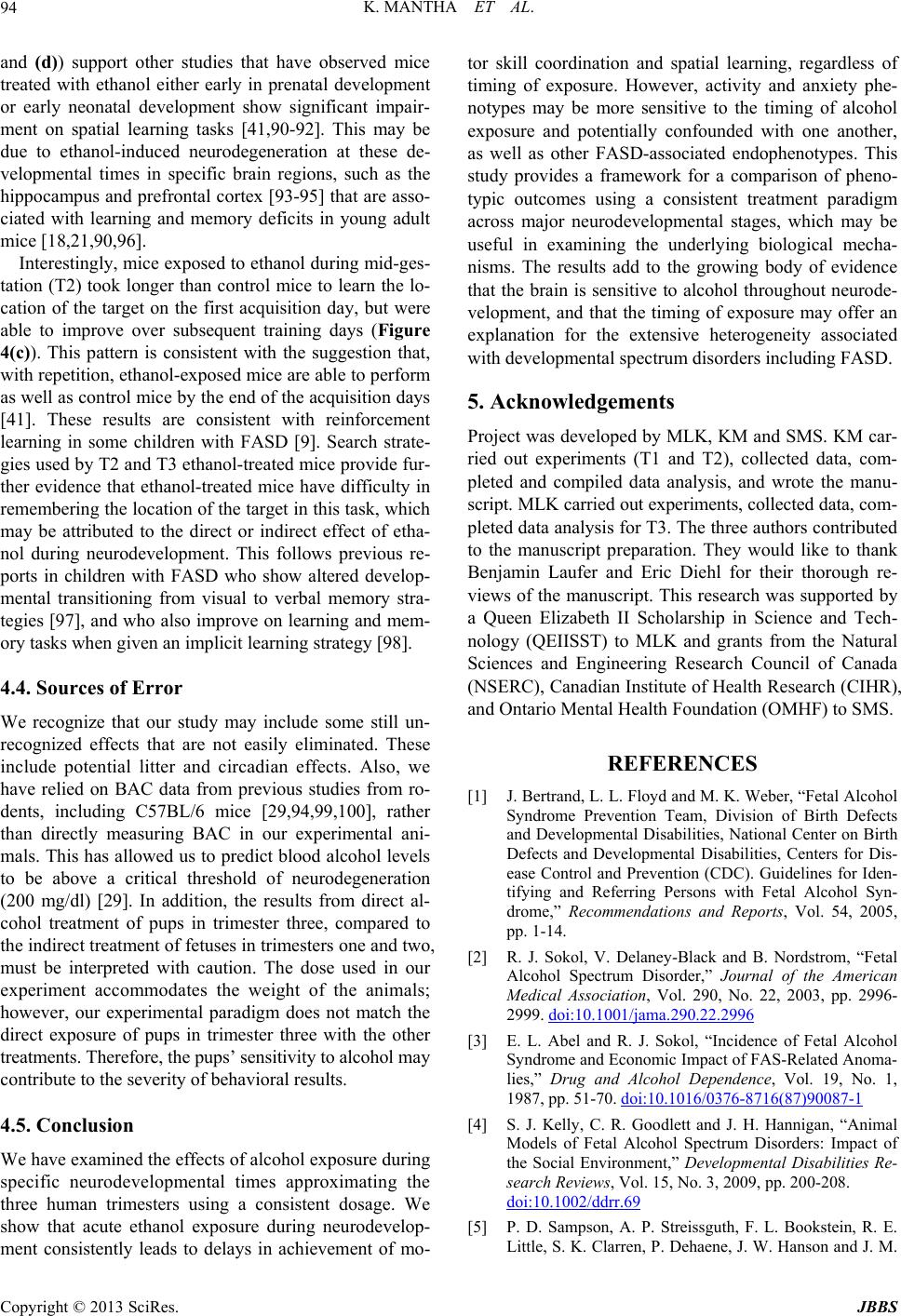 K. MANTHA ET AL. 94 and (d)) support other studies that have observed mice treated with ethanol either early in prenatal development or early neonatal development show significant impair- ment on spatial learning tasks [41,90-92]. This may be due to ethanol-induced neurodegeneration at these de- velopmental times in specific brain regions, such as the hippocampus and prefrontal cortex [93-95] that are asso- ciated with learning and memory deficits in young adult mice [18,21,90,96]. Interestingly, mice exposed to ethanol during mid-ges- tation (T2) took longer than control mice to learn the lo- cation of the target on the first acquisition day, but were able to improve over subsequent training days (Figure 4(c)). This pattern is consistent with the suggestion that, with repetition, ethanol-exposed mice are able to perform as well as control mice by the end of the acquisition days [41]. These results are consistent with reinforcement learning in some children with FASD [9]. Search strate- gies used by T2 and T3 ethanol-treated mice provide fur- ther evidence that ethanol-treated mice have difficulty in remembering the location of the target in this task, which may be attributed to the direct or indirect effect of etha- nol during neurodevelopment. This follows previous re- ports in children with FASD who show altered develop- mental transitioning from visual to verbal memory stra- tegies [97], and who also improve on learning and mem- ory tasks when given an implicit learning strategy [98]. 4.4. Sources of Error We recognize that our study may include some still un- recognized effects that are not easily eliminated. These include potential litter and circadian effects. Also, we have relied on BAC data from previous studies from ro- dents, including C57BL/6 mice [29,94,99,100], rather than directly measuring BAC in our experimental ani- mals. This has allowed us to predict blood alcohol levels to be above a critical threshold of neurodegeneration (200 mg/dl) [29]. In addition, the results from direct al- cohol treatment of pups in trimester three, compared to the indirect treatment of fetuses in trimesters one and two, must be interpreted with caution. The dose used in our experiment accommodates the weight of the animals; however, our experimental paradigm does not match the direct exposure of pups in trimester three with the other treatments. Therefore, the pups’ sensitivity to alcohol may contribute to the severity of behavioral results. 4.5. Conclusion We have examined the effects of alcohol exposure during specific neurodevelopmental times approximating the three human trimesters using a consistent dosage. We show that acute ethanol exposure during neurodevelop- ment consistently leads to delays in achievement of mo- tor skill coordination and spatial learning, regardless of timing of exposure. However, activity and anxiety phe- notypes may be more sensitive to the timing of alcohol exposure and potentially confounded with one another, as well as other FASD-associated endophenotypes. This study provides a framework for a comparison of pheno- typic outcomes using a consistent treatment paradigm across major neurodevelopmental stages, which may be useful in examining the underlying biological mecha- nisms. The results add to the growing body of evidence that the brain is sensitive to alcohol throughout neurode- velopment, and that the timing of exposure may offer an explanation for the extensive heterogeneity associated with developmental spectrum disorders including FASD. 5. Acknowledgements Project was developed by MLK, KM and SMS. KM car- ried out experiments (T1 and T2), collected data, com- pleted and compiled data analysis, and wrote the manu- script. MLK carried out experiments, collected data, com- pleted data analysis for T3. The three authors contributed to the manuscript preparation. They would like to thank Benjamin Laufer and Eric Diehl for their thorough re- views of the manuscript. This research was supported by a Queen Elizabeth II Scholarship in Science and Tech- nology (QEIISST) to MLK and grants from the Natural Sciences and Engineering Research Council of Canada (NSERC), Canadian Institute of Health Research (CIHR), and Ontario Mental Health Foundation (OMHF) to SMS. REFERENCES [1] J. Bertrand, L. L. Floyd and M. K. Weber, “Fetal Alcohol Syndrome Prevention Team, Division of Birth Defects and Developmental Disabilities, National Center on Birth Defects and Developmental Disabilities, Centers for Dis- ease Control and Prevention (CDC). Guidelines for Iden- tifying and Referring Persons with Fetal Alcohol Syn- drome,” Recommendations and Reports, Vol. 54, 2005, pp. 1-14. [2] R. J. Sokol, V. Delaney-Black and B. Nordstrom, “Fetal Alcohol Spectrum Disorder,” Journal of the American Medical Association, Vol. 290, No. 22, 2003, pp. 2996- 2999. doi:10.1001/jama.290.22.2996 [3] E. L. Abel and R. J. Sokol, “Incidence of Fetal Alcohol Syndrome and Economic Impact of FAS-Related Anoma- lies,” Drug and Alcohol Dependence, Vol. 19, No. 1, 1987, pp. 51-70. doi:10.1016/0376-8716(87)90087-1 [4] S. J. Kelly, C. R. Goodlett and J. H. Hannigan, “Animal Models of Fetal Alcohol Spectrum Disorders: Impact of the Social Environment,” Developmental Disabilities Re- search Reviews, Vol. 15, No. 3, 2009, pp. 200-208. doi:10.1002/ddrr.69 [5] P. D. Sampson, A. P. Streissguth, F. L. Bookstein, R. E. Little, S. K. Clarren, P. Dehaene, J. W. Hanson and J. M. Copyright © 2013 SciRes. JBBS
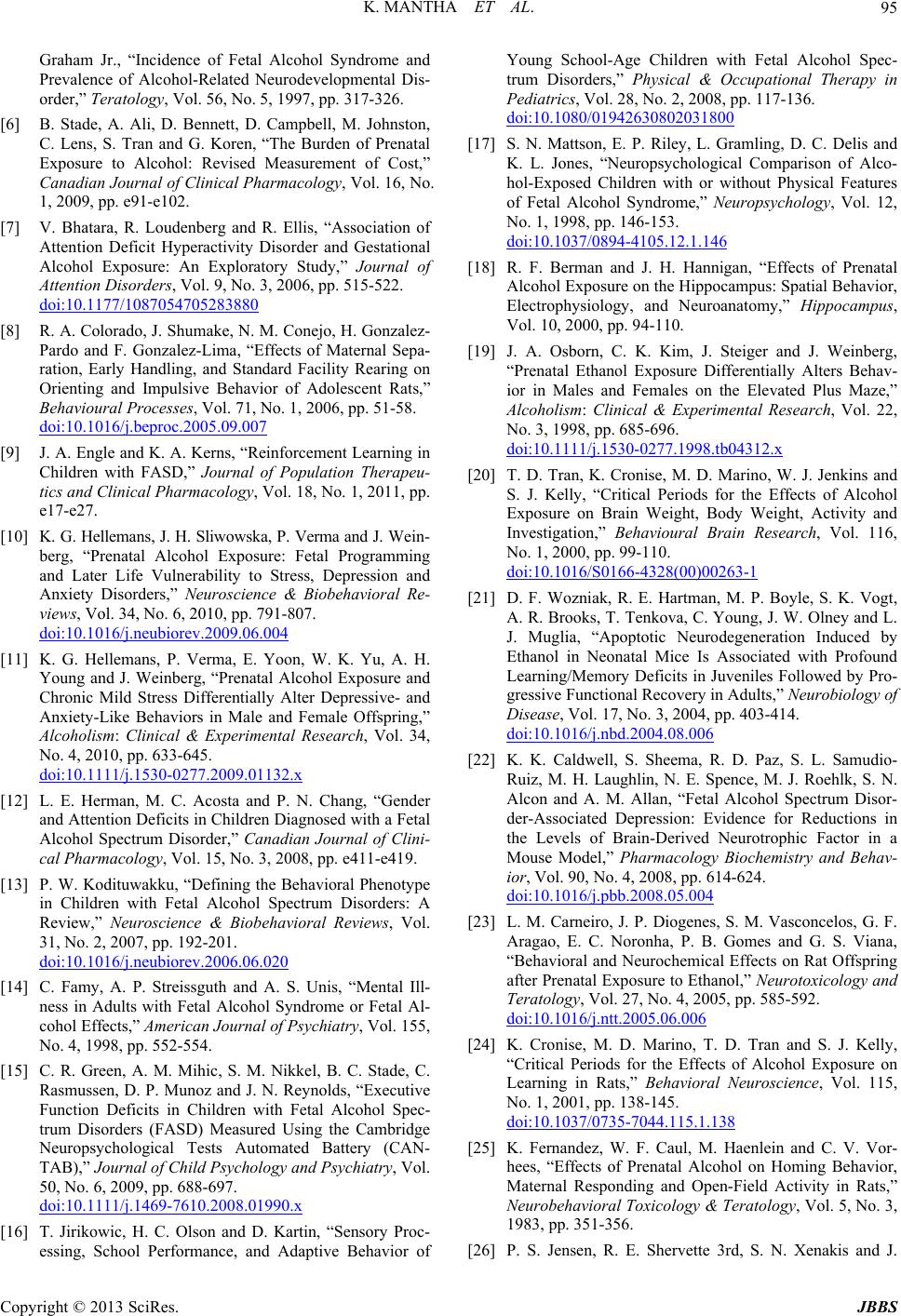 K. MANTHA ET AL. 95 Graham Jr., “Incidence of Fetal Alcohol Syndrome and Prevalence of Alcohol-Related Neurodevelopmental Dis- order,” Teratology, Vol. 56, No. 5, 1997, pp. 317-326. [6] B. Stade, A. Ali, D. Bennett, D. Campbell, M. Johnston, C. Lens, S. Tran and G. Koren, “The Burden of Prenatal Exposure to Alcohol: Revised Measurement of Cost,” Canadian Journal of Clinical Pharmacology, Vol. 16, No. 1, 2009, pp. e91-e102. [7] V. Bhatara, R. Loudenberg and R. Ellis, “Association of Attention Deficit Hyperactivity Disorder and Gestational Alcohol Exposure: An Exploratory Study,” Journal of Attention Disorders, Vol. 9, No. 3, 2006, pp. 515-522. doi:10.1177/1087054705283880 [8] R. A. Colorado, J. Shumake, N. M. Conejo, H. Gonzalez- Pardo and F. Gonzalez-Lima, “Effects of Maternal Sepa- ration, Early Handling, and Standard Facility Rearing on Orienting and Impulsive Behavior of Adolescent Rats,” Behavioural Processes, Vol. 71, No. 1, 2006, pp. 51-58. doi:10.1016/j.beproc.2005.09.007 [9] J. A. Engle and K. A. Kerns, “Reinforcement Learning in Children with FASD,” Journal of Population Therapeu- tics and Clinical Pharmacology, Vol. 18, No. 1, 2011, pp. e17-e27. [10] K. G. Hellemans, J. H. Sliwowska, P. Verma and J. Wein- berg, “Prenatal Alcohol Exposure: Fetal Programming and Later Life Vulnerability to Stress, Depression and Anxiety Disorders,” Neuroscience & Biobehavioral Re- views, Vol. 34, No. 6, 2010, pp. 791-807. doi:10.1016/j.neubiorev.2009.06.004 [11] K. G. Hellemans, P. Verma, E. Yoon, W. K. Yu, A. H. Young and J. Weinberg, “Prenatal Alcohol Exposure and Chronic Mild Stress Differentially Alter Depressive- and Anxiety-Like Behaviors in Male and Female Offspring,” Alcoholism: Clinical & Experimental Research, Vol. 34, No. 4, 2010, pp. 633-645. doi:10.1111/j.1530-0277.2009.01132.x [12] L. E. Herman, M. C. Acosta and P. N. Chang, “Gender and Attention Deficits in Children Diagnosed with a Fetal Alcohol Spectrum Disorder,” Canadian Journal of Clini- cal Pharmacology, Vol. 15, No. 3, 2008, pp. e411-e419. [13] P. W. Kodituwakku, “Defining the Behavioral Phenotype in Children with Fetal Alcohol Spectrum Disorders: A Review,” Neuroscience & Biobehavioral Reviews, Vol. 31, No. 2, 2007, pp. 192-201. doi:10.1016/j.neubiorev.2006.06.020 [14] C. Famy, A. P. Streissguth and A. S. Unis, “Mental Ill- ness in Adults with Fetal Alcohol Syndrome or Fetal Al- cohol Effects,” American Journal of Psychiatry, Vol. 155, No. 4, 1998, pp. 552-554. [15] C. R. Green, A. M. Mihic, S. M. Nikkel, B. C. Stade, C. Rasmussen, D. P. Munoz and J. N. Reynolds, “Executive Function Deficits in Children with Fetal Alcohol Spec- trum Disorders (FASD) Measured Using the Cambridge Neuropsychological Tests Automated Battery (CAN- TAB),” Journal of Child Psychology and Psychiatry, Vol. 50, No. 6, 2009, pp. 688-697. doi:10.1111/j.1469-7610.2008.01990.x [16] T. Jirikowic, H. C. Olson and D. Kartin, “Sensory Proc- essing, School Performance, and Adaptive Behavior of Young School-Age Children with Fetal Alcohol Spec- trum Disorders,” Physical & Occupational Therapy in Pediatrics, Vol. 28, No. 2, 2008, pp. 117-136. doi:10.1080/01942630802031800 [17] S. N. Mattson, E. P. Riley, L. Gramling, D. C. Delis and K. L. Jones, “Neuropsychological Comparison of Alco- hol-Exposed Children with or without Physical Features of Fetal Alcohol Syndrome,” Neuropsychology, Vol. 12, No. 1, 1998, pp. 146-153. doi:10.1037/0894-4105.12.1.146 [18] R. F. Berman and J. H. Hannigan, “Effects of Prenatal Alcohol Exposure on the Hippocampus: Spatial Behavior, Electrophysiology, and Neuroanatomy,” Hippocampus, Vol. 10, 2000, pp. 94-110. [19] J. A. Osborn, C. K. Kim, J. Steiger and J. Weinberg, “Prenatal Ethanol Exposure Differentially Alters Behav- ior in Males and Females on the Elevated Plus Maze,” Alcoholism: Clinical & Experimental Research, Vol. 22, No. 3, 1998, pp. 685-696. doi:10.1111/j.1530-0277.1998.tb04312.x [20] T. D. Tran, K. Cronise, M. D. Marino, W. J. Jenkins and S. J. Kelly, “Critical Periods for the Effects of Alcohol Exposure on Brain Weight, Body Weight, Activity and Investigation,” Behavioural Brain Research, Vol. 116, No. 1, 2000, pp. 99-110. doi:10.1016/S0166-4328(00)00263-1 [21] D. F. Wozniak, R. E. Hartman, M. P. Boyle, S. K. Vogt, A. R. Brooks, T. Tenkova, C. Young, J. W. Olney and L. J. Muglia, “Apoptotic Neurodegeneration Induced by Ethanol in Neonatal Mice Is Associated with Profound Learning/Memory Deficits in Juveniles Followed by Pro- gressive Functional Recovery in Adults,” Neurobiology of Disease, Vol. 17, No. 3, 2004, pp. 403-414. doi:10.1016/j.nbd.2004.08.006 [22] K. K. Caldwell, S. Sheema, R. D. Paz, S. L. Samudio- Ruiz, M. H. Laughlin, N. E. Spence, M. J. Roehlk, S. N. Alcon and A. M. Allan, “Fetal Alcohol Spectrum Disor- der-Associated Depression: Evidence for Reductions in the Levels of Brain-Derived Neurotrophic Factor in a Mouse Model,” Pharmacology Biochemistry and Behav- ior, Vol. 90, No. 4, 2008, pp. 614-624. doi:10.1016/j.pbb.2008.05.004 [23] L. M. Carneiro, J. P. Diogenes, S. M. Vasconcelos, G. F. Aragao, E. C. Noronha, P. B. Gomes and G. S. Viana, “Behavioral and Neurochemical Effects on Rat Offspring after Prenatal Exposure to Ethanol,” Neurotoxicology and Teratology, Vol. 27, No. 4, 2005, pp. 585-592. doi:10.1016/j.ntt.2005.06.006 [24] K. Cronise, M. D. Marino, T. D. Tran and S. J. Kelly, “Critical Periods for the Effects of Alcohol Exposure on Learning in Rats,” Behavioral Neuroscience, Vol. 115, No. 1, 2001, pp. 138-145. doi:10.1037/0735-7044.115.1.138 [25] K. Fernandez, W. F. Caul, M. Haenlein and C. V. Vor- hees, “Effects of Prenatal Alcohol on Homing Behavior, Maternal Responding and Open-Field Activity in Rats,” Neurobehavioral Toxicology & Teratology, Vol. 5, No. 3, 1983, pp. 351-356. [26] P. S. Jensen, R. E. Shervette 3rd, S. N. Xenakis and J. Copyright © 2013 SciRes. JBBS
 K. MANTHA ET AL. 96 Richters, “Anxiety and Depressive Disorders in Attention Deficit Disorder with Hyperactivity: New Findings,” American Journal of Psychiatry, Vol. 150, No. 8, 1993, pp. 1203-1209. [27] M. J. O’Connor and B. Paley, “The Relationship of Pre- natal Alcohol Exposure and the Postnatal Environment to Child Depressive Symptoms,” Journal of Pediatric Psy- chology, Vol. 31, No. 1, 2006, pp. 50-64. doi:10.1093/jpepsy/jsj021 [28] J. Dobbing and J. Sands, “Comparative Aspects of the Brain Growth Spurt,” Early Human Development, Vol. 3, No. 1, 1979, pp. 79-83. doi:10.1016/0378-3782(79)90022-7 [29] C. Ikonomidou, P. Bittigau, M. J. Ishimaru, D. F. Woz- niak, C. Koch, K. Genz, M. T. Price, V. Stefovska, F. Hor- ster, T. Tenkova, K. Dikranian and J. W. Olney, “Etha- nol-Induced Apoptotic Neurodegeneration and Fetal Al- cohol Syndrome,” Science, Vol. 287, No. 5455, 2000, pp. 1056-1060. doi:10.1126/science.287.5455.1056 [30] K. L. Howdeshell, “A Model of the Development of the Brain as a Construct of the Thyroid System,” Environ- mental Health Perspectives, Vol. 110, No. 3, 2002, pp. 337-348. doi:10.1289/ehp.02110s3337 [31] S. Bake, J. D. Tingling and R. C. Miranda, “Ethanol Ex- posure during Pregnancy Persistently Attenuates Cra- nially Directed Blood Flow in the Developing Fetus: Evidence from Ultrasound Imaging in a Murine Second Trimester Equivalent Model,” Alcoholism: Clinical and Experimental Research, Vol. 36, No. 5, 2011, pp. 748- 758. doi:10.1111/j.1530-0277.2011.01676.x [32] C. Guerri, A. Bazinet and E. P. Riley, “Foetal Alcohol Spectrum Disorders and Alterations in Brain and Behav- iour,” Alcohol, Vol. 44, No. 2, 2009, pp. 108-114. doi:10.1093/alcalc/agn105 [33] J. R. West, S. J. Kelly and D. R. Pierce, “Severity of Al- cohol-Induced Deficits in Rats during the Third Trimester Equivalent Is Determined by the Pattern of Exposure,” Alcohol, Vol. 1, 1987, pp. 461-465. [34] R. Caetano, S. Ramisetty-Mikler, L. R. Floyd and C. Mc- Grath, “The Epidemiology of Drinking among Women of Child-Bearing Age,” Alcoholism: Clinical and Experi- mental Research, Vol. 30, No. 6, 2006, pp. 1023-1030. doi:10.1111/j.1530-0277.2006.00116.x [35] H. A. Flynn, S. M. Marcus, K. L. Barry and F. C. Blow, “Rates and Correlates of Alcohol Use among Pregnant Women in Obstetrics Clinics,” Alcoholism: Clinical and Experimental Research, Vol. 27, No. 1, 2003, pp. 81-87. [36] A. Malassine, J. L. Frendo and D. Evain-Brion, “A Com- parison of Placental Development and Endocrine Func- tions between the Human and Mouse Model,” Human Reproduction Update, Vol. 9, No. 6, 2003, pp. 531-539. doi:10.1093/humupd/dmg043 [37] B. Zhang, X. Wang and A. J. Nazarali, “Ascorbic Acid Reverses Valproic Acid-Induced Inhibition of Hoxa2 and Maintains Glutathione Homeostasis in Mouse Embryos in Culture,” Cellular and Molecular Neurobiology, Vol. 30, No. 1, 2010, pp. 137-148. doi:10.1007/s10571-009-9438-7 [38] J. De Miranda, K. Yaddanapudi, M. Hornig, G. Villar, R. Serge and W. I. Lipkin, “Induction of Toll-Like Receptor 3-Mediated Immunity during Gestation Inhibits Cortical Neurogenesis and Causes Behavioral Disturbances,” MBio, Vol. 1, No. 4, 2010, Article ID: e00176-10. doi:10.1128/mBio.00176-10 [39] T. Levav, T. Saar, L. Berkovich and H. Golan, “Perinatal Exposure to GABA-Transaminase Inhibitor Impaired Psy- chomotor Function in the Developing and Adult Mouse,” International Journal of Developmental Neuroscience, Vol. 22, No. 3, 2004, pp. 137-147. doi:10.1016/j.ijdevneu.2004.03.004 [40] J. M. Hill, M. A. Lim and M. M. Stone, “Developmental Milestones in the Newborn Mouse,” In: I. Gozes, Ed., Neuropeptide Techniques, Humana Press, Totowa, 2008, p. 149. doi:10.1007/978-1-60327-099-1_10 [41] M. L. Kleiber, E. Wright and S. M. Singh, “Maternal Voluntary Drinking in C57BL/6J Mice: Advancing a Model for Fetal Alcohol Spectrum Disorders,” Behav- ioural Brain Research, Vol. 223, No. 2, 2011, pp. 376- 387. doi:10.1016/j.bbr.2011.05.005 [42] J. Clarkson and A. E. Herbison, “Postnatal Development of Kisspeptin Neurons in Mouse Hypothalamus; Sexual Dimorphism and Projections to Gonadotropin-Releasing Hormone Neurons,” Endocrinology, Vol. 147, No. 12, 2006, pp. 5817-5825. doi:10.1210/en.2006-0787 [43] D. D. Langleben, G. Austin, G. Krikorian, H. W. Ridle- huber, M. L. Goris and H. W. Strauss, “Interhemispheric Asymmetry of Regional Cerebral Blood Flow in Prepu- bescent Boys with Attention Deficit Hyperactivity Disor- der,” Nuclear Medicine Communications, Vol. 22, No. 12, 2001, pp. 1333-1340. doi:10.1097/00006231-200112000-00009 [44] N. D. Volkow, S. J. Gatley, J. S. Fowler, G. J. Wang and J. Swanson, “Serotonin and the Therapeutic Effects of Ri- talin,” Science, Vol. 299, No. 5463, 2000, p. 11. doi:10.1126/science.288.5463.11a [45] J. N. Crawley and R. Paylor, “A Proposed Test Battery and Constellations of Specific Behavioral Paradigms to Investigate the Behavioral Phenotypes of Transgenic and Knockout Mice,” Hormones and Behavior, Vol. 31, No. 3, 1997, pp. 197-211. doi:10.1006/hbeh.1997.1382 [46] B. Sunyer, S. Patil, H. Hoger and G. Lubec, “Barnes Maze, a Useful Task to Assess Spatial Reference Memory in the Mice,” Nature Protocols, 2007. doi:10.1038/nprot.2007.390 [47] F. E. Harrison, R. S. Reiserer, A. J. Tomarken and M. P. McDonald, “Spatial and Nonspatial Escape Strategies in the Barnes Maze,” Learning & Memory, Vol. 13, No. 6, 2006, pp. 809-819. doi:10.1101/lm.334306 [48] T. P. O’Leary, V. Savoie and R. E. Brown, “Learning, Memory and Search Strategies of Inbred Mouse Strains with Different Visual Abilities in the Barnes Maze,” Be- havioural Brain Research, Vol. 216, No. 2, 2011, pp. 531-542. doi:10.1016/j.bbr.2010.08.030 [49] H. C. Becker and C. L. Randall, “Effects of Prenatal Etha- nol Exposure in C57BL Mice on Locomotor Activity and Passive Avoidance Behavior,” Psychopharmacology, Vol. 97, No. 1, 1989, pp. 40-44. doi:10.1007/BF00443410 [50] R. E. Brown, “Behavioural Phenotyping of Transgenic Copyright © 2013 SciRes. JBBS
 K. MANTHA ET AL. 97 Mice,” Canadian Journal of Experimental Psychology, Vol. 61, No. 4, 2007, pp. 328-344. doi:10.1037/cjep2007033 [51] S. L. Inman-Wood, M. T. Williams, L. L. Morford and C. V. Vorhees, “Effects of Prenatal Cocaine on Morris and Barnes Maze Tests of Spatial Learning and Memory in the Offspring of C57BL/6J Mice,” Neurotoxicology and Teratology, Vol. 22, No. 4, 2000, pp. 547-557. doi:10.1016/S0892-0362(00)00084-2 [52] K. L. McIlwain, M. Y. Merriweather, L. A. Yuva-Paylor and R. Paylor, “The Use of Behavioral Test Batteries: Ef- fects of Training History,” Physiology & Behavior, Vol. 73, No. 5, 2001, pp. 705-717. doi:10.1016/S0031-9384(01)00528-5 [53] P. N. Pompl, M. J. Mullan, K. Bjugstad and G. W. Aren- dash, “Adaptation of the Circular Platform Spatial Mem- ory Task for Mice: Use in Detecting Cognitive Impair- ment in the APP(SW) Transgenic Mouse Model for Alz- heimer’s Disease,” Journal of Neuroscience Methods, Vol. 87, No. 1, 1999, pp. 87-95. doi:10.1016/S0165-0270(98)00169-1 [54] W. O. Kalberg, B. Provost, S. J. Tollison, B. G. Tabach- nick, L. K. Robinson, H. Eugene Hoyme, P. M. Trujillo, D. Buckley, A. S. Aragon and P. A. May, “Comparison of Motor Delays in Young Children with Fetal Alcohol Syn- drome to Those with Prenatal Alcohol Exposure and with No Prenatal Alcohol Exposure,” Alcoholism: Clinical and Experimental Research, Vol. 30, No. 12, 2006, pp. 2037- 2045. doi:10.1111/j.1530-0277.2006.00250.x [55] N. L. Staisey and P. A. Fried, “Relationships between Mo- derate Maternal Alcohol Consumption during Pregnancy and Infant Neurological Development,” Journal of Stud- ies on Alcohol, Vol. 44, No. 2, 1983, pp. 262-270. [56] J. Altman and K. Sudarshan, “Postnatal Development of Locomotion in the Laboratory Rat,” Animal Behaviour, Vol. 23, No. 4, 1975, pp. 896-920. doi:10.1016/0003-3472(75)90114-1 [57] D. J. Bonthius and J. R. West, “Alcohol-Induced Neu- ronal Loss in Developing Rats: Increased Brain Damage with Binge Exposure,” Alcoholism: Clinical and Experi- mental Research, Vol. 14, No. 1, 1990, pp. 107-118. doi:10.1111/j.1530-0277.1990.tb00455.x [58] M. W. Burke, R. M. Palmour, F. R. Ervin and M. Ptito, “Neuronal Reduction in Frontal Cortex of Primates after Prenatal Alcohol Exposure,” Neuroreport, Vol. 20, No. 1, 2009, pp. 13-17. doi:10.1097/WNR.0b013e32831b449c [59] T. A. Cudd, W. J. Chen, S. E. Parnell and J. R. West, “Third Trimester Binge Ethanol Exposure Results in Fetal Hypercapnea and Acidemia but Not Hypoxemia in Preg- nant Sheep,” Alcoholism: Clinical and Experimental Re- search, Vol. 25, No. 2, 2001, pp. 269-276. doi:10.1111/j.1530-0277.2001.tb02208.x [60] L. E. Kotch and K. K. Sulik, “Experimental Fetal Alcohol Syndrome: Proposed Pathogenic Basis for a Variety of Associated Facial and Brain Anomalies,” American Jour- nal of Medical Genetics, Vol. 44, No. 2, 1992, pp. 168- 176. doi:10.1002/ajmg.1320440210 [61] D. E. Mayock, D. Ness, R. L. Mondares and C. A. Glea- son, “Binge Alcohol Exposure in the Second Trimester Attenuates Fetal Cerebral Blood Flow Response to Hypo- xia,” Journal of Applied Physiology, Vol. 102, No. 3, 2007, pp. 972-977. doi:10.1152/japplphysiol.00956.2006 [62] L. Mrzljak, H. B. Uylings, I. Kostovic and C. G. Van Eden, “Prenatal Development of Neurons in the Human Prefrontal Cortex: I. A Qualitative Golgi Study,” Journal of Comparative Neurology, Vol. 271, No. 3, 1988, pp. 355-386. doi:10.1002/cne.902710306 [63] A. Diamond, “Close Interrelation of Motor Development and Cognitive Development and of the Cerebellum and Prefrontal Cortex,” Child Development, Vol. 71, No. 1, 2000, pp. 44-56. doi:10.1111/1467-8624.00117 [64] M. Matsumura, N. Sadato, T. Kochiyama, S. Nakamura, E. Naito, K. Matsunami, R. Kawashima, H. Fukuda and Y. Yonekura, “Role of the Cerebellum in Implicit Motor Skill Learning: A PET Study,” Brain Research Bulletin, Vol. 63, No. 6, 2004, pp. 471-483. doi:10.1016/j.brainresbull.2004.04.008 [65] J. R. West and K. M. Hamre, “Effects of Alcohol Expo- sure during Different Periods of Development: Changes in Hippocampal Mossy Fibers,” Brain Research, Vol. 349, 1985, pp. 280-284. [66] K. L. Jones, D. W. Smith, C. N. Ulleland and P. Streiss- guth, “Pattern of Malformation in Offspring of Chronic Alcoholic Mothers,” Lancet, Vol. 301, No. 7815, 1973, pp. 1267-1271. doi:10.1016/S0140-6736(73)91291-9 [67] K. L. Jones, “The Effects of Alcohol on Fetal Develop- ment,” Birth Defects Research Part C: Embryo Today, Vol. 93, No. 1, 2011, pp. 3-11. [68] L. Kooistra, B. Ramage, S. Crawford, M. Cantell, S. Wormsbecker, B. Gibbard and B. J. Kaplan, “Can Atten- tion Deficit Hyperactivity Disorder and Fetal Alcohol Spectrum Disorder Be Differentiated by Motor and Bal- ance Deficits?” Human Movement Science, Vol. 28, No. 4, 2009, pp. 529-542. doi:10.1016/j.humov.2009.01.007 [69] H. C. Steinhausen, J. Willms, C. W. Metzke and H. L. Spohr, “Behavioural Phenotype in Foetal Alcohol Syn- drome and Foetal Alcohol Effects,” Developmental Me- dicine & Child Neurology, Vol. 45, No. 3, 2003, pp. 179- 182. doi:10.1111/j.1469-8749.2003.tb00927.x [70] A. P. Streissguth, H. M. Barr, D. C. Martin and C. S. Her- man, “Effects of Maternal Alcohol, Nicotine, and Caf- feine Use during Pregnancy on Infant Mental and Motor Development at Eight Months,” Alcoholism: Clinical and Experimental Research, Vol. 4, No. 2, 1980, pp. 152-164. doi:10.1111/j.1530-0277.1980.tb05630.x [71] H. C. Leiner, A. L. Leiner and R. S. Dow, “The Human Cerebro-Cerebellar System: Its Computing, Cognitive, and Language Skills,” Behavioural Brain Research, Vol. 44, No. 2, 1991, pp. 113-128. doi:10.1016/S0166-4328(05)80016-6 [72] K. Dikranian, Y. Q. Qin, J. Labruyere, B. Nemmers and J. W. Olney, “Ethanol-Induced Neuroapoptosis in the Deve- loping Rodent Cerebellum and Related Brain Stem Struc- tures,” Developmental Brain Research, Vol. 155, No. 1, 2005, pp. 1-13. doi:10.1016/j.devbrainres.2004.11.005 [73] C. Downing, C. Balderrama-Durbin, J. Hayes, T. E. John- son and D. Gilliam, “No Effect of Prenatal Alcohol Ex- posure on Activity in Three Inbred Strains of Mice,” Al- Copyright © 2013 SciRes. JBBS
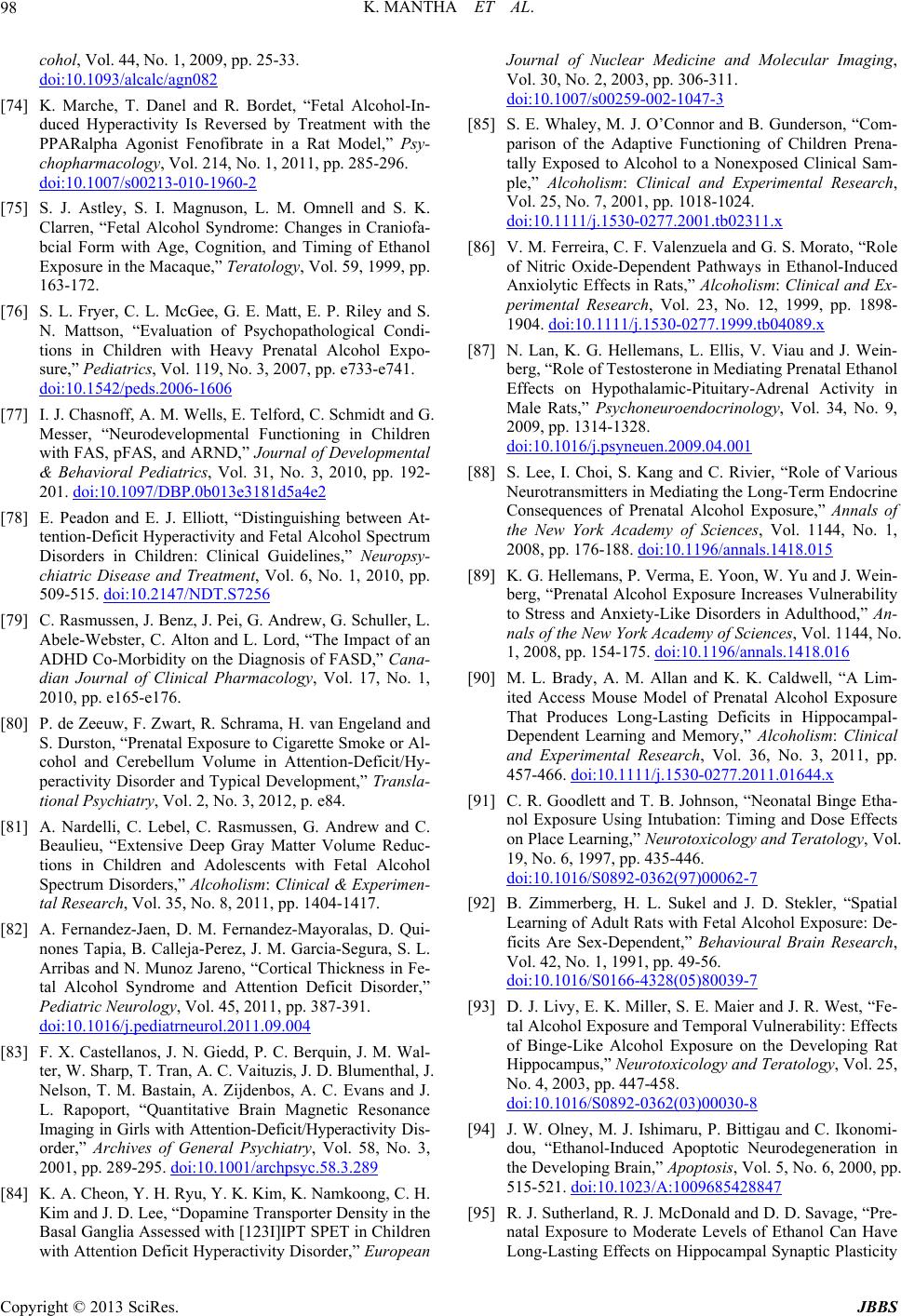 K. MANTHA ET AL. 98 cohol, Vol. 44, No. 1, 2009, pp. 25-33. doi:10.1093/alcalc/agn082 [74] K. Marche, T. Danel and R. Bordet, “Fetal Alcohol-In- duced Hyperactivity Is Reversed by Treatment with the PPARalpha Agonist Fenofibrate in a Rat Model,” Psy- chopharmacology, Vol. 214, No. 1, 2011, pp. 285-296. doi:10.1007/s00213-010-1960-2 [75] S. J. Astley, S. I. Magnuson, L. M. Omnell and S. K. Clarren, “Fetal Alcohol Syndrome: Changes in Craniofa- bcial Form with Age, Cognition, and Timing of Ethanol Exposure in the Macaque,” Teratology, Vol. 59, 1999, pp. 163-172. [76] S. L. Fryer, C. L. McGee, G. E. Matt, E. P. Riley and S. N. Mattson, “Evaluation of Psychopathological Condi- tions in Children with Heavy Prenatal Alcohol Expo- sure,” Pediatrics, Vol. 119, No. 3, 2007, pp. e733-e741. doi:10.1542/peds.2006-1606 [77] I. J. Chasnoff, A. M. Wells, E. Telford, C. Schmidt and G. Messer, “Neurodevelopmental Functioning in Children with FAS, pFAS, and ARND,” Journal of Developmental & Behavioral Pediatrics, Vol. 31, No. 3, 2010, pp. 192- 201. doi:10.1097/DBP.0b013e3181d5a4e2 [78] E. Peadon and E. J. Elliott, “Distinguishing between At- tention-Deficit Hyperactivity and Fetal Alcohol Spectrum Disorders in Children: Clinical Guidelines,” Neuropsy- chiatric Disease and Treatment, Vol. 6, No. 1, 2010, pp. 509-515. doi:10.2147/NDT.S7256 [79] C. Rasmussen, J. Benz, J. Pei, G. Andrew, G. Schuller, L. Abele-Webster, C. Alton and L. Lord, “The Impact of an ADHD Co-Morbidity on the Diagnosis of FASD,” Cana- dian Journal of Clinical Pharmacology, Vol. 17, No. 1, 2010, pp. e165-e176. [80] P. de Zeeuw, F. Zwart, R. Schrama, H. van Engeland and S. Durston, “Prenatal Exposure to Cigarette Smoke or Al- cohol and Cerebellum Volume in Attention-Deficit/Hy- peractivity Disorder and Typical Development,” Transla- tional Psychiatry, Vol. 2, No. 3, 2012, p. e84. [81] A. Nardelli, C. Lebel, C. Rasmussen, G. Andrew and C. Beaulieu, “Extensive Deep Gray Matter Volume Reduc- tions in Children and Adolescents with Fetal Alcohol Spectrum Disorders,” Alcoholism: Clinical & Experimen- tal Research, Vol. 35, No. 8, 2011, pp. 1404-1417. [82] A. Fernandez-Jaen, D. M. Fernandez-Mayoralas, D. Qui- nones Tapia, B. Calleja-Perez, J. M. Garcia-Segura, S. L. Arribas and N. Munoz Jareno, “Cortical Thickness in Fe- tal Alcohol Syndrome and Attention Deficit Disorder,” Pediatric Neurology, Vol. 45, 2011, pp. 387-391. doi:10.1016/j.pediatrneurol.2011.09.004 [83] F. X. Castellanos, J. N. Giedd, P. C. Berquin, J. M. Wal- ter, W. Sharp, T. Tran, A. C. Vaituzis, J. D. Blumenthal, J. Nelson, T. M. Bastain, A. Zijdenbos, A. C. Evans and J. L. Rapoport, “Quantitative Brain Magnetic Resonance Imaging in Girls with Attention-Deficit/Hyperactivity Dis- order,” Archives of General Psychiatry, Vol. 58, No. 3, 2001, pp. 289-295. doi:10.1001/archpsyc.58.3.289 [84] K. A. Cheon, Y. H. Ryu, Y. K. Kim, K. Namkoong, C. H. Kim and J. D. Lee, “Dopamine Transporter Density in the Basal Ganglia Assessed with [123I]IPT SPET in Children with Attention Deficit Hyperactivity Disorder,” European Journal of Nuclear Medicine and Molecular Imaging, Vol. 30, No. 2, 2003, pp. 306-311. doi:10.1007/s00259-002-1047-3 [85] S. E. Whaley, M. J. O’Connor and B. Gunderson, “Com- parison of the Adaptive Functioning of Children Prena- tally Exposed to Alcohol to a Nonexposed Clinical Sam- ple,” Alcoholism: Clinical and Experimental Research, Vol. 25, No. 7, 2001, pp. 1018-1024. doi:10.1111/j.1530-0277.2001.tb02311.x [86] V. M. Ferreira, C. F. Valenzuela and G. S. Morato, “Role of Nitric Oxide-Dependent Pathways in Ethanol-Induced Anxiolytic Effects in Rats,” Alcoholism: Clinical and Ex- perimental Research, Vol. 23, No. 12, 1999, pp. 1898- 1904. doi:10.1111/j.1530-0277.1999.tb04089.x [87] N. Lan, K. G. Hellemans, L. Ellis, V. Viau and J. Wein- berg, “Role of Testosterone in Mediating Prenatal Ethanol Effects on Hypothalamic-Pituitary-Adrenal Activity in Male Rats,” Psychoneuroendocrinology, Vol. 34, No. 9, 2009, pp. 1314-1328. doi:10.1016/j.psyneuen.2009.04.001 [88] S. Lee, I. Choi, S. Kang and C. Rivier, “Role of Various Neurotransmitters in Mediating the Long-Term Endocrine Consequences of Prenatal Alcohol Exposure,” Annals of the New York Academy of Sciences, Vol. 1144, No. 1, 2008, pp. 176-188. doi:10.1196/annals.1418.015 [89] K. G. Hellemans, P. Verma, E. Yoon, W. Yu and J. Wein- berg, “Prenatal Alcohol Exposure Increases Vulnerability to Stress and Anxiety-Like Disorders in Adulthood,” An- nals of the New York Academy of Sciences, Vol. 1144, No. 1, 2008, pp. 154-175. doi:10.1196/annals.1418.016 [90] M. L. Brady, A. M. Allan and K. K. Caldwell, “A Lim- ited Access Mouse Model of Prenatal Alcohol Exposure That Produces Long-Lasting Deficits in Hippocampal- Dependent Learning and Memory,” Alcoholism: Clinical and Experimental Research, Vol. 36, No. 3, 2011, pp. 457-466. doi:10.1111/j.1530-0277.2011.01644.x [91] C. R. Goodlett and T. B. Johnson, “Neonatal Binge Etha- nol Exposure Using Intubation: Timing and Dose Effects on Place Learning,” Neurotoxicology and Teratology, Vol. 19, No. 6, 1997, pp. 435-446. doi:10.1016/S0892-0362(97)00062-7 [92] B. Zimmerberg, H. L. Sukel and J. D. Stekler, “Spatial Learning of Adult Rats with Fetal Alcohol Exposure: De- ficits Are Sex-Dependent,” Behavioural Brain Research, Vol. 42, No. 1, 1991, pp. 49-56. doi:10.1016/S0166-4328(05)80039-7 [93] D. J. Livy, E. K. Miller, S. E. Maier and J. R. West, “Fe- tal Alcohol Exposure and Temporal Vulnerability: Effects of Binge-Like Alcohol Exposure on the Developing Rat Hippocampus,” Neurotoxicology and Teratology, Vol. 25, No. 4, 2003, pp. 447-458. doi:10.1016/S0892-0362(03)00030-8 [94] J. W. Olney, M. J. Ishimaru, P. Bittigau and C. Ikonomi- dou, “Ethanol-Induced Apoptotic Neurodegeneration in the Developing Brain,” Apoptosis, Vol. 5, No. 6, 2000, pp. 515-521. doi:10.1023/A:1009685428847 [95] R. J. Sutherland, R. J. McDonald and D. D. Savage, “Pre- natal Exposure to Moderate Levels of Ethanol Can Have Long-Lasting Effects on Hippocampal Synaptic Plasticity Copyright © 2013 SciRes. JBBS
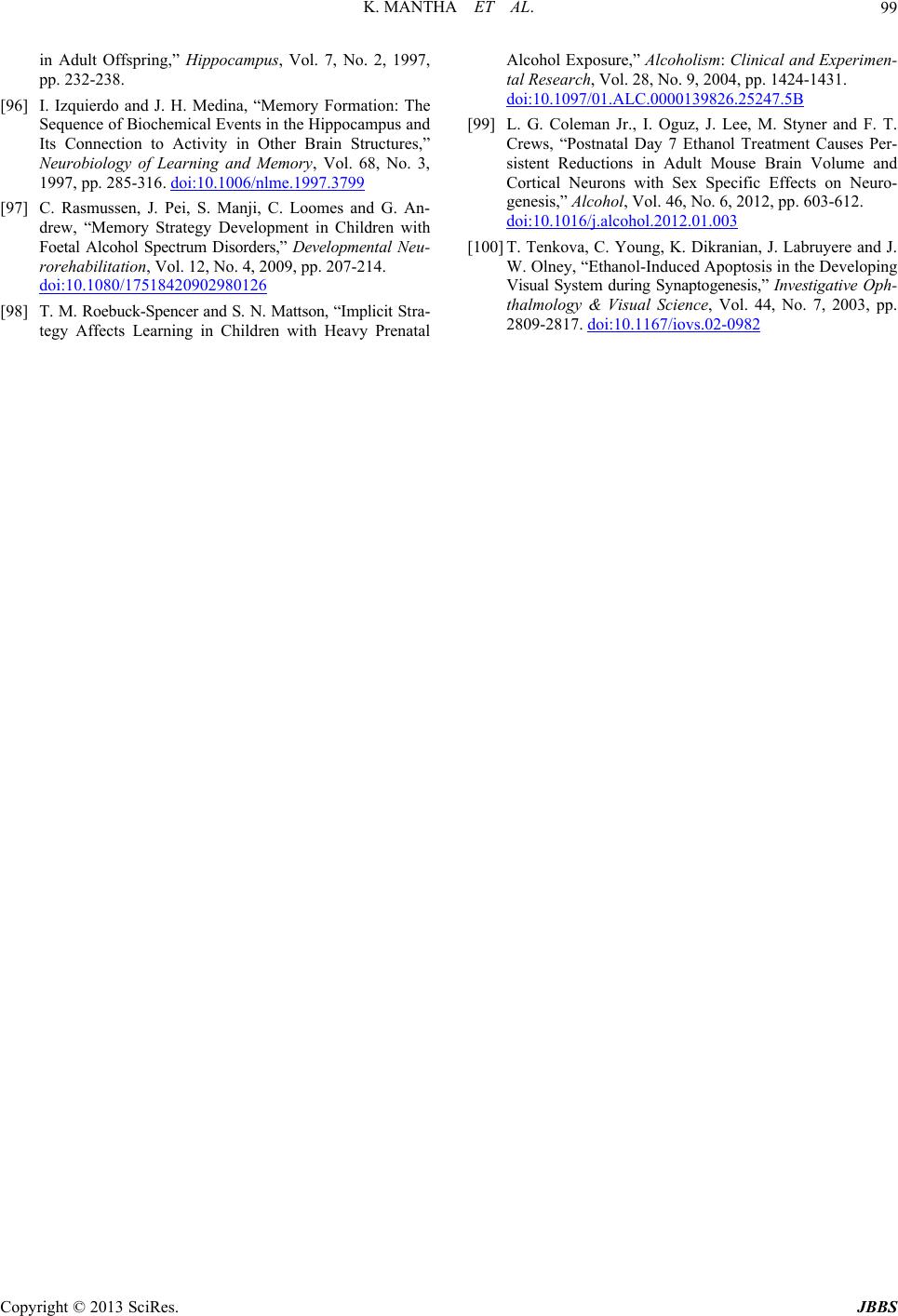 K. MANTHA ET AL. Copyright © 2013 SciRes. JBBS 99 in Adult Offspring,” Hippocampus, Vol. 7, No. 2, 1997, pp. 232-238. [96] I. Izquierdo and J. H. Medina, “Memory Formation: The Sequence of Biochemical Events in the Hippocampus and Its Connection to Activity in Other Brain Structures,” Neurobiology of Learning and Memory, Vol. 68, No. 3, 1997, pp. 285-316. doi:10.1006/nlme.1997.3799 [97] C. Rasmussen, J. Pei, S. Manji, C. Loomes and G. An- drew, “Memory Strategy Development in Children with Foetal Alcohol Spectrum Disorders,” Developmental Neu- rorehabilitation, Vol. 12, No. 4, 2009, pp. 207-214. doi:10.1080/17518420902980126 [98] T. M. Roebuck-Spencer and S. N. Mattson, “Implicit Stra- tegy Affects Learning in Children with Heavy Prenatal Alcohol Exposure,” Alcoholism: Clinical and Experimen- tal Research, Vol. 28, No. 9, 2004, pp. 1424-1431. doi:10.1097/01.ALC.0000139826.25247.5B [99] L. G. Coleman Jr., I. Oguz, J. Lee, M. Styner and F. T. Crews, “Postnatal Day 7 Ethanol Treatment Causes Per- sistent Reductions in Adult Mouse Brain Volume and Cortical Neurons with Sex Specific Effects on Neuro- genesis,” Alcohol, Vol. 46, No. 6, 2012, pp. 603-612. doi:10.1016/j.alcohol.2012.01.003 [100] T. Tenkova, C. Young, K. Dikranian, J. Labruyere and J. W. Olney, “Ethanol-Induced Apoptosis in the Developing Visual System during Synaptogenesis,” Investigative Oph- thalmology & Visual Science, Vol. 44, No. 7, 2003, pp. 2809-2817. doi:10.1167/iovs.02-0982
|