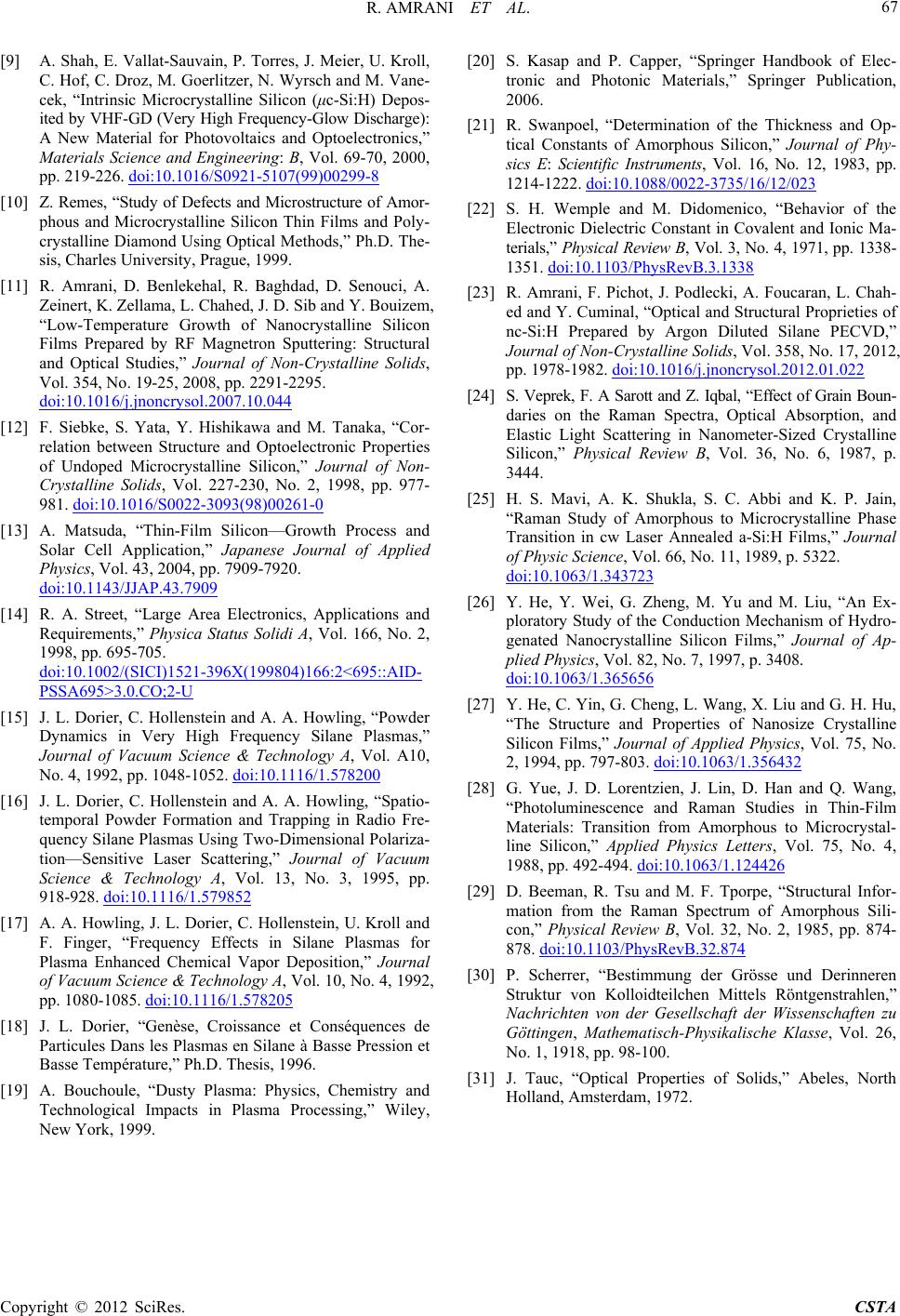
R. AMRANI ET AL.
Copyright © 2012 SciRes. CSTA
67
[9] A. Shah, E. Vallat-Sauvain, P. Torres, J. Meier, U. Kroll,
C. Hof, C. Droz, M. Goerlitzer, N. Wyrsch and M. Vane-
cek, “Intrinsic Microcrystalline Silicon (μc-Si:H) Depos-
ited by VHF-GD (Very High Frequency-Glow Discharge):
A New Material for Photovoltaics and Optoelectronics,”
Materials Science and Engineering: B, Vol. 69-70, 2000,
pp. 219-226. doi:10.1016/S0921-5107(99)00299-8
[10] Z. Remes, “Study of Defects and Microstructure of Amor-
phous and Microcrystalline Silicon Thin Films and Poly-
crystalline Diamond Using Optical Methods,” Ph.D. The-
sis, Charles University, Prague, 1999.
[11] R. Amrani, D. Benlekehal, R. Baghdad, D. Senouci, A.
Zeinert, K. Zellama, L. Chahed, J. D. Sib and Y. Bouizem,
“Low-Temperature Growth of Nanocrystalline Silicon
Films Prepared by RF Magnetron Sputtering: Structural
and Optical Studies,” Journal of Non-Crystalline Solids,
Vol. 354, No. 19-25, 2008, pp. 2291-2295.
doi:10.1016/j.jnoncrysol.2007.10.044
[12] F. Siebke, S. Yata, Y. Hishikawa and M. Tanaka, “Cor-
relation between Structure and Optoelectronic Properties
of Undoped Microcrystalline Silicon,” Journal of Non-
Crystalline Solids, Vol. 227-230, No. 2, 1998, pp. 977-
981. doi:10.1016/S0022-3093(98)00261-0
[13] A. Matsuda, “Thin-Film Silicon—Growth Process and
Solar Cell Application,” Japanese Journal of Applied
Physics, Vol. 43, 2004, pp. 7909-7920.
doi:10.1143/JJAP.43.7909
[14] R. A. Street, “Large Area Electronics, Applications and
Requirements,” Physica Status Solidi A, Vol. 166, No. 2,
1998, pp. 695-705.
doi:10.1002/(SICI)1521-396X(199804)166:2<695::AID-
PSSA695>3.0.CO;2-U
[15] J. L. Dorier, C. Hollenstein and A. A. Howling, “Powder
Dynamics in Very High Frequency Silane Plasmas,”
Journal of Vacuum Science & Technology A, Vol. A10,
No. 4, 1992, pp. 1048-1052. doi:10.1116/1.578200
[16] J. L. Dorier, C. Hollenstein and A. A. Howling, “Spatio-
temporal Powder Formation and Trapping in Radio Fre-
quency Silane Plasmas Using Two-Dimensional Polariza-
tion—Sensitive Laser Scattering,” Journal of Vacuum
Science & Technology A, Vol. 13, No. 3, 1995, pp.
918-928. doi:10.1116/1.579852
[17] A. A. Howling, J. L. Dorier, C. Hollenstein, U. Kroll and
F. Finger, “Frequency Effects in Silane Plasmas for
Plasma Enhanced Chemical Vapor Deposition,” Journal
of Vacuum Science & Technology A, Vol. 10, No. 4, 1992,
pp. 1080-1085. doi:10.1116/1.578205
[18] J. L. Dorier, “Genèse, Croissance et Conséquences de
Particules Dans les Plasmas en Silane à Basse Pression et
Basse Température,” Ph.D. Thesis, 1996.
[19] A. Bouchoule, “Dusty Plasma: Physics, Chemistry and
Technological Impacts in Plasma Processing,” Wiley,
New York, 1999.
[20] S. Kasap and P. Capper, “Springer Handbook of Elec-
tronic and Photonic Materials,” Springer Publication,
2006.
[21] R. Swanpoel, “Determination of the Thickness and Op-
tical Constants of Amorphous Silicon,” Journal of Phy-
sics E: Scientific Instruments, Vol. 16, No. 12, 1983, pp.
1214-1222. doi:10.1088/0022-3735/16/12/023
[22] S. H. Wemple and M. Didomenico, “Behavior of the
Electronic Dielectric Constant in Covalent and Ionic Ma-
terials,” Physical Review B, Vol. 3, No. 4, 1971, pp. 1338-
1351. doi:10.1103/PhysRevB.3.1338
[23] R. Amrani, F. Pichot, J. Podlecki, A. Foucaran, L. Chah-
ed and Y. Cuminal, “Optical and Structural Proprieties of
nc-Si:H Prepared by Argon Diluted Silane PECVD,”
Journal of Non-Crystalline Solids, Vol. 358, No. 17, 2012,
pp. 1978-1982. doi:10.1016/j.jnoncrysol.2012.01.022
[24] S. Veprek, F. A Sarott and Z. Iqbal, “Effect of Grain Boun-
daries on the Raman Spectra, Optical Absorption, and
Elastic Light Scattering in Nanometer-Sized Crystalline
Silicon,” Physical Review B, Vol. 36, No. 6, 1987, p.
3444.
[25] H. S. Mavi, A. K. Shukla, S. C. Abbi and K. P. Jain,
“Raman Study of Amorphous to Microcrystalline Phase
Transition in cw Laser Annealed a-Si:H Films,” Journal
of Physic Science, Vol. 66, No. 11, 1989, p. 5322.
doi:10.1063/1.343723
[26] Y. He, Y. Wei, G. Zheng, M. Yu and M. Liu, “An Ex-
ploratory Study of the Conduction Mechanism of Hydro-
genated Nanocrystalline Silicon Films,” Journal of Ap-
plied Physics, Vol. 82, No. 7, 1997, p. 3408.
doi:10.1063/1.365656
[27] Y. He, C. Yin, G. Cheng, L. Wang, X. Liu and G. H. Hu,
“The Structure and Properties of Nanosize Crystalline
Silicon Films,” Journal of Applied Physics, Vol. 75, No.
2, 1994, pp. 797-803. doi:10.1063/1.356432
[28] G. Yue, J. D. Lorentzien, J. Lin, D. Han and Q. Wang,
“Photoluminescence and Raman Studies in Thin-Film
Materials: Transition from Amorphous to Microcrystal-
line Silicon,” Applied Physics Letters, Vol. 75, No. 4,
1988, pp. 492-494. doi:10.1063/1.124426
[29] D. Beeman, R. Tsu and M. F. Tporpe, “Structural Infor-
mation from the Raman Spectrum of Amorphous Sili-
con,” Physical Review B, Vol. 32, No. 2, 1985, pp. 874-
878. doi:10.1103/PhysRevB.32.874
[30] P. Scherrer, “Bestimmung der Grösse und Derinneren
Struktur von Kolloidteilchen Mittels Röntgenstrahlen,”
Nachrichten von der Gesellschaft der Wissenschaften zu
Göttingen, Mathematisch-Physikalische Klasse, Vol. 26,
No. 1, 1918, pp. 98-100.
[31] J. Tauc, “Optical Properties of Solids,” Abeles, North
Holland, Amsterdam, 1972.