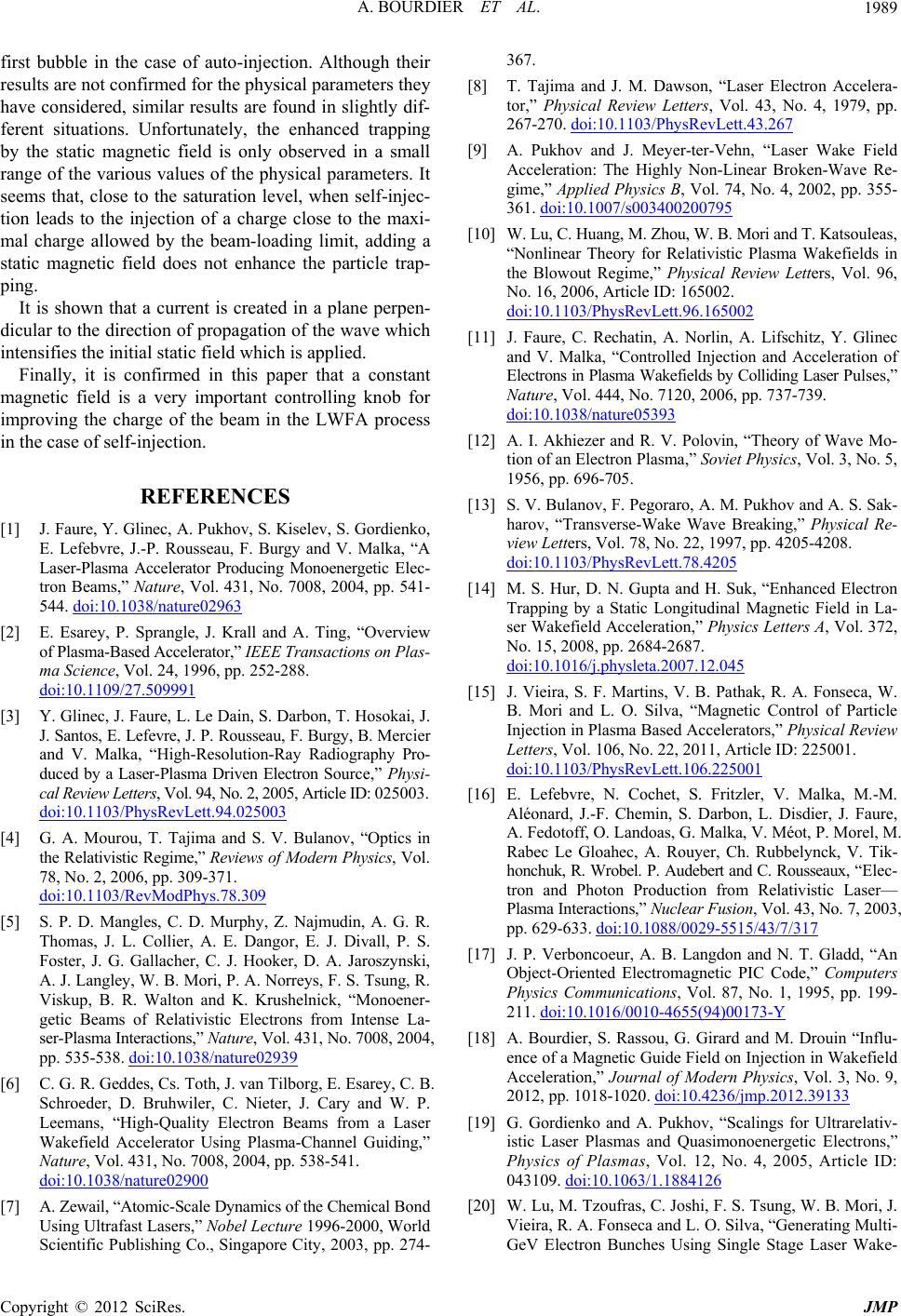
A. BOURDIER ET AL. 1989
first bubble in the case of auto-injection. Although their
results are not confirmed for the physical parameters they
have considered, similar results are found in slightly dif-
ferent situations. Unfortunately, the enhanced trapping
by the static magnetic field is only observed in a small
range of the various values of the physical parameters. It
seems that, close to the saturation level, when self-injec-
tion leads to the injection of a charge close to the maxi-
mal charge allowed by the beam-loading limit, adding a
static magnetic field does not enhance the particle trap-
ping.
It is shown that a current is created in a plane perpen-
dicular to the direction of propagation of the wave which
intensifies the initial static field which is applied.
Finally, it is confirmed in this paper that a constant
magnetic field is a very important controlling knob for
improving the charge of the beam in the LWFA process
in the case of self-injection.
REFERENCES
[1] J. Faure, Y. Glinec, A. Pukhov, S. Kiselev, S. Gordienko,
E. Lefebvre, J.-P. Rousseau, F. Burgy and V. Malka, “A
Laser-Plasma Accelerator Producing Monoenergetic Elec-
tron Beams,” Nature, Vol. 431, No. 7008, 2004, pp. 541-
544. doi:10.1038/nature02963
[2] E. Esarey, P. Sprangle, J. Krall and A. Ting, “Overview
of Plasma-Based Accelerator,” IEEE Transactions on Plas-
ma Science, Vol. 24, 1996, pp. 252-288.
doi:10.1109/27.509991
[3] Y. Glinec, J. Faure, L. Le Dain, S. Darbon, T. Hosokai, J.
J. Santos, E. Lefevre, J. P. Rousseau, F. Burgy, B. Mercier
and V. Malka, “High-Resolution-Ray Radiography Pro-
duced by a Laser-Plasma Driven Electron Source,” Physi-
cal Review Letters, Vol. 94, No. 2, 2005, Article ID: 025003.
doi:10.1103/PhysRevLett.94.025003
[4] G. A. Mourou, T. Tajima and S. V. Bulanov, “Optics in
the Relativistic Regime,” Reviews of Modern Physics, Vol.
78, No. 2, 2006, pp. 309-371.
doi:10.1103/RevModPhys.78.309
[5] S. P. D. Mangles, C. D. Murphy, Z. Najmudin, A. G. R.
Thomas, J. L. Collier, A. E. Dangor, E. J. Divall, P. S.
Foster, J. G. Gallacher, C. J. Hooker, D. A. Jaroszynski,
A. J. Langley, W. B. Mori, P. A. Norreys, F. S. Tsung, R.
Viskup, B. R. Walton and K. Krushelnick, “Monoener-
getic Beams of Relativistic Electrons from Intense La-
ser-Plasma Interactions,” Nature, Vol. 431, No. 7008, 2004,
pp. 535-538. doi:10.1038/nature02939
[6] C. G. R. Geddes, Cs. Toth, J. van Tilborg, E. Esarey, C. B.
Schroeder, D. Bruhwiler, C. Nieter, J. Cary and W. P.
Leemans, “High-Quality Electron Beams from a Laser
Wakefield Accelerator Using Plasma-Channel Guiding,”
Nature, Vol. 431, No. 7008, 2004, pp. 538-541.
doi:10.1038/nature02900
[7] A. Zewail, “Atomic-Scale Dynamics of the Chemical Bond
Using Ultrafast Lasers,” Nobel Lecture 1996-2000, World
Scientific Publishing Co., Singapore City, 2003, pp. 274-
367.
[8] T. Tajima and J. M. Dawson, “Laser Electron Accelera-
tor,” Physical Review Letters, Vol. 43, No. 4, 1979, pp.
267-270. doi:10.1103/PhysRevLett.43.267
[9] A. Pukhov and J. Meyer-ter-Vehn, “Laser Wake Field
Acceleration: The Highly Non-Linear Broken-Wave Re-
gime,” Applied Physics B, Vol. 74, No. 4, 2002, pp. 355-
361. doi:10.1007/s003400200795
[10] W. Lu, C. Huang, M. Zhou, W. B. Mori and T. Katsouleas,
“Nonlinear Theory for Relativistic Plasma Wakefields in
the Blowout Regime,” Physical Review Letters, Vol. 96,
No. 16, 2006, Article ID: 165002.
doi:10.1103/PhysRevLett.96.165002
[11] J. Faure, C. Rechatin, A. Norlin, A. Lifschitz, Y. Glinec
and V. Malka, “Controlled Injection and Acceleration of
Electrons in Plasma Wakefields by Colliding Laser Pulses,”
Nature, Vol. 444, No. 7120, 2006, pp. 737-739.
doi:10.1038/nature05393
[12] A. I. Akhiezer and R. V. Polovin, “Theory of Wave Mo-
tion of an Electron Plasma,” Soviet Physics, Vol. 3, No. 5,
1956, pp. 696-705.
[13] S. V. Bulanov, F. Pegoraro, A. M. Pukhov and A. S. Sak-
harov, “Transverse-Wake Wave Breaking,” Physical Re-
view Letters, Vol. 78, No. 22, 1997, pp. 4205-4208.
doi:10.1103/PhysRevLett.78.4205
[14] M. S. Hur, D. N. Gupta and H. Suk, “Enhanced Electron
Trapping by a Static Longitudinal Magnetic Field in La-
ser Wakefield Acceleration,” Physics Letters A, Vol. 372,
No. 15, 2008, pp. 2684-2687.
doi:10.1016/j.physleta.2007.12.045
[15] J. Vieira, S. F. Martins, V. B. Pathak, R. A. Fonseca, W.
B. Mori and L. O. Silva, “Magnetic Control of Particle
Injection in Plasma Based Accelerators,” Physical Review
Letters, Vol. 106, No. 22, 2011, Article ID: 225001.
doi:10.1103/PhysRevLett.106.225001
[16] E. Lefebvre, N. Cochet, S. Fritzler, V. Malka, M.-M.
Aléonard, J.-F. Chemin, S. Darbon, L. Disdier, J. Faure,
A. Fedotoff, O. Landoas, G. Malka, V. Méot, P. Morel, M.
Rabec Le Gloahec, A. Rouyer, Ch. Rubbelynck, V. Tik-
honchuk, R. Wrobel. P. Audebert and C. Rousseaux, “Elec-
tron and Photon Production from Relativistic Laser—
Plasma Interactions,” Nuclear Fusion, Vol. 43, No. 7, 2003,
pp. 629-633. doi:10.1088/0029-5515/43/7/317
[17] J. P. Verboncoeur, A. B. Langdon and N. T. Gladd, “An
Object-Oriented Electromagnetic PIC Code,” Computers
Physics Communications, Vol. 87, No. 1, 1995, pp. 199-
211. doi:10.1016/0010-4655(94)00173-Y
[18] A. Bourdier, S. Rassou, G. Girard and M. Drouin “Influ-
ence of a Magnetic Guide Field on Injection in Wakefield
Acceleration,” Journal of Modern Physics, Vol. 3, No. 9,
2012, pp. 1018-1020. doi:10.4236/jmp.2012.39133
[19] G. Gordienko and A. Pukhov, “Scalings for Ultrarelativ-
istic Laser Plasmas and Quasimonoenergetic Electrons,”
Physics of Plasmas, Vol. 12, No. 4, 2005, Article ID:
043109. doi:10.1063/1.1884126
[20] W. Lu, M. Tzoufras, C. Joshi, F. S. Tsung, W. B. Mori, J.
Vieira, R. A. Fonseca and L. O. Silva, “Generating Multi-
GeV Electron Bunches Using Single Stage Laser Wake-
Copyright © 2012 SciRes. JMP