 Atmospheric and Climate Sciences, 2012, 2, 501-509 http://dx.doi.org/10.4236/acs.2012.24044 Published Online October 2012 (http://www.SciRP.org/journal/acs) The Effect of Catena Position on Greenho use Gas Emissions from Dambo Located Termite (Odontotermes transvaalensis) Mounds from Central Zimbabwe George Nyamadzawo1,2*, Jephita Gotosa1, Justice Muvengwi1, Menas Wuta2, Justice Nyamangara3, Philip Nyamugafata2, Jeff L. Smith4 1Department of Environmental Science, Bindura University of Science Education, Bindura, Zimbabwe 2Department of Soil Science and Agricultural Engineering, University of Zimbabwe, Mount Pleasant, Harare, Zimbabwe 3International Centre for Research in Semi Arid Tropics (ICRISAT) Matopos, Bulawayo, Zimbabwe 4USDA-Agricultural Research Service, Washington DC, USA Email: *gnyama@yahoo.com Received June 11, 2012; revised July 13, 2012; accepted July 25, 2012 ABSTRACT Methane (CH4), carbon dioxide (CO2) and nitrous oxide (N2O) are greenhouse gases (GHGs) which cause global warming. Natural sources of GHGs include wetlands and termites. Previous studies have quantified GHG emissions from upland termites and no study has reported GHG emissions from seasonal wetlands (dambo) located termite mounds. The objective of this study was to evaluate the effect of dambo catena position on termite mound distribution and GHG emissions. It was hypothesized that mound density and GHG emissions from Odontotermes transvaalensis mounds, vary with catena position. The evaluated catena positions were margin, mid-slope, lower slope and bottom. Mound density was significantly lower in the bottom when compared to the other catena positions. The mean GHG fluxes were 88 µg·m2·hr–1, 0.78 mg m–2·hr–1 and 1361 mg·m–2·hr–1 for N2O, CH4 and CO2 respectively. Fluxes varied with catena position and were 0.48, 0.72, 1.35 and 0.79 mg·m–2·hr–1 for CH4, and 1173.7, 1440.7, 1798.7 and 922.8 mg· m–2·hr–1 for CO2 in the margin, mid-slope, lower slope and the bottom catena position respectively. For N2O, there were no significant differences between catena positions. It was concluded that dambo located Odontotermes trans- vaalensis termite mounds are an important source of GHGs, and emissions varied with catena position for CO2 and CH4. Keywords: Greenhouse Gas Emissions; Termites Mounds; Odontotermes transvaalensis; Dambos; Catena Position 1. Introduction Greenhouse gases (GHGs) are among the major causes of global warming [1]. The greenhouse effect occurs when greenhouse gases; methane (CH4), carbon dioxide (CO2) and nitrous oxide (N2O) trap long wavelength radiation that is reflected from the earth’s surface, hence affecting the balance of heat radiation through the entire atmosphere, resulting in rising temperatures. Analysis has shown that atmospheric concentrations of methane have increased by approximately 145 percent since 1800 [2] and the CH4 atmospheric mixing ratios have incr- eased by 2.5 times, reaching 1750 ppbv (parts per billion by volume) in 2001 [3]. Among the three major GHGs, N2O has a bigger global warming potential (GWP). On a mol- ecule for molecule basis, the GWP of N2O are 310 and 21 times more, than that of CO2 and CH4 respectively, over a 100 year time scale [1]. The residence time of N2O is 115 years compared to 100 years for CO2 whilst the ability to absorb infra red radiation is 216 greater than that of CO2 [4]. Thus, N2O is known for its long lasting greenhouse gas effect. Sources of GHGs maybe anthropogenic e.g. rice pad- dies or natural e.g. wetlands [3,5,6]. Current estimates for CH4 emissions from wetlands are between 20 and 25% of the global annual emissions [6] and of this, 60% is from tropical wetlands. Central and Southern African regions are occupied by some of the largest seasonal wetlands in Africa. These seasonal wetlands which are commonly called dambos constitute the largest geographic extent of seasonal wetlands on the elevated plateaus of Africa [7,8]. Dambos are seasonally saturated, grassy, gently slop- ing valley floors [7,9,10], which are conservatively esti- mated at 20% of the gently undulating land surface of the Central African Plateau [7]. In Zimbabwe dambos cover an estimated 1.28 million hactares (ha) of land. Dambos are mainly used as pastures for livestock and for gardens *Corresponding author. C opyright © 2012 SciRes. ACS
 G. NYAMADZAWO ET AL. 502 in the smallholder farming sector. Dambos from Zimb- abwe are normally divided into five basic soil-vege- tation-topography units (catena positions) namely upland, margin; mid-slope; lower-slope and bottoms [11]. The general trend observed when moving from upland to bot- tom catena in areas underlain by mafic parent material, is an increase in soil organic matter (SOM) content [12-14] and an increase in clay contents. The clay content incr- eases as a result of depositions of clay that is eroded from the margins and upland. The clay mineralogy in dambos may also change from non active clays in the margin, to active clays in the bottoms. The gently sloping landscapes of dambos are interru- pted by mounds which are formed by termites, Odonto- termes transvaalensis [15] and are characterized by drier soils and frequently covered by trees [14,16]. Several Odontotermes species are common in the moist savannas of southern Africa, and species are specific to soil mois- ture regimes and to certain soil types [15]. Dambos of southern Africa are occupied by the Odontotermes trans- vaalensis species, and estimates are that they contain mil- lions of termite mounds which are scattered throughout these gently undulating floors. Odontotermes transva- alensis species favour areas that are found throughout the central watershed of Zimbabwe with high water table, drainage lines and seasonally water logged areas (dam- bos) [15,17]. Dambos are mainly used as pastures, and the termite mounds provide good forage as they are always covered by grasses because of the high fertility of soils of the mounds [15]. Odontoterme transvaalensis build large termite mounds with a vent at the center. The mound maintains an equitable set of moisture and temperature conditions within the hive. Termite mound densities may be 10 mounds ha–1 ([18], while Schuurman and Dangerfield [17] reported densities of between 0.05 and 6 mounds per hectare (ha) in the seasonally flooded Okavango delta. In dambos not all mounds contain term- ites as some are abandoned. Termite mounds can also be categorized on the basis of activity, either as active or none active. Active mounds are those mounds which contain termites while inactive mounds are those that have been abandoned by termites. The proportions of live mounds are variable in dambos, for example, in southern Nigeria, [19] reported 60% live mounds for some species. Although termite populations are active in the middle latitude environments, the highest concentration of mou- nds and nests are found in the tropical regions of Africa, Asia, Australia and South America which contribute ap- proximately 80% of global termite mound emissions [20]. The role of termites in global carbon cycle in the savan- nas of Africa have been reported by several authors e.g. [20-26]. However, few studies have quantified termite mound densities in dambos e.g. [27] and [18]. The role of dambos termite on carbon and nitrogen cycling and GHG emissions from central and southern Africa has been overlooked and is much less understood. From the available literature there is no reported data on GHG emissions from organic matter rich dambo located ter- mite mounds from Zimbabwe, or the region which is covered by millions of hectares of dambos which contain millions of termite mounds. The few studies that have reported GHG emissions from termite mounds have fo- cused mainly at GHG fluxes from upland e.g. [24,25,28]. This study is reporting the first dataset on GHG emis- sions from dambos located Odontotermes transvaalensis mounds from Zimbabwe. The objectives of the study were to quantify the effect of the catena position on ter- mite mound density and on GHG emissions. It was hy- pothesized that termite mound densities vary with catena position and that GHG emissions from dambo located termite mounds also vary with catena position. 2. Methodology 2.1. Study Sites The University of Zimbabwe Farm (UZF) is located 20 km North of Harare along the Mazowe road (GPS loca- tion 17˚43'S, 31˚00'E), (Figure 1). The UZF is in Natu- ral Region IIa with an annual rainfall varying from 700 - 1000 mm which is received between November and April. The mean annual temperature ranges from 16 to 20˚C [29]. The farm is located on a dolerite terrain and the site has heavy-textured soils (red clay; Chromic luvisol). The soil changes from a red colour in well drained areas to grey and black in the dambos. The sea- sonally waterlogged dambos at the UZF are mainly used as pastures for livestock and are surrounded in uplands by maize, soya bean fields and miombo woodlands. Gas OTSWANA OUTH AFRICA AMBIA OZAMBIQ Kilometers 0 50 100 150 31º 1 8 º 31º 1 8 º HARARE Bindur a Marondera Rusape M utare ational capital City Main r oa d Bulawayo Hwange Lupa ne Gwanda Zvis hav ane Gweru Kado ma Plumtree Masvingo Shuri g wi Mvuma Sh amva Mt. Darwin Be Study area OZAMBIQUE t b ridge Figure 1. The Zimbabwe map showing the study site, Uni- versity of Zimbabwe Farm (UZF). Copyright © 2012 SciRes. ACS
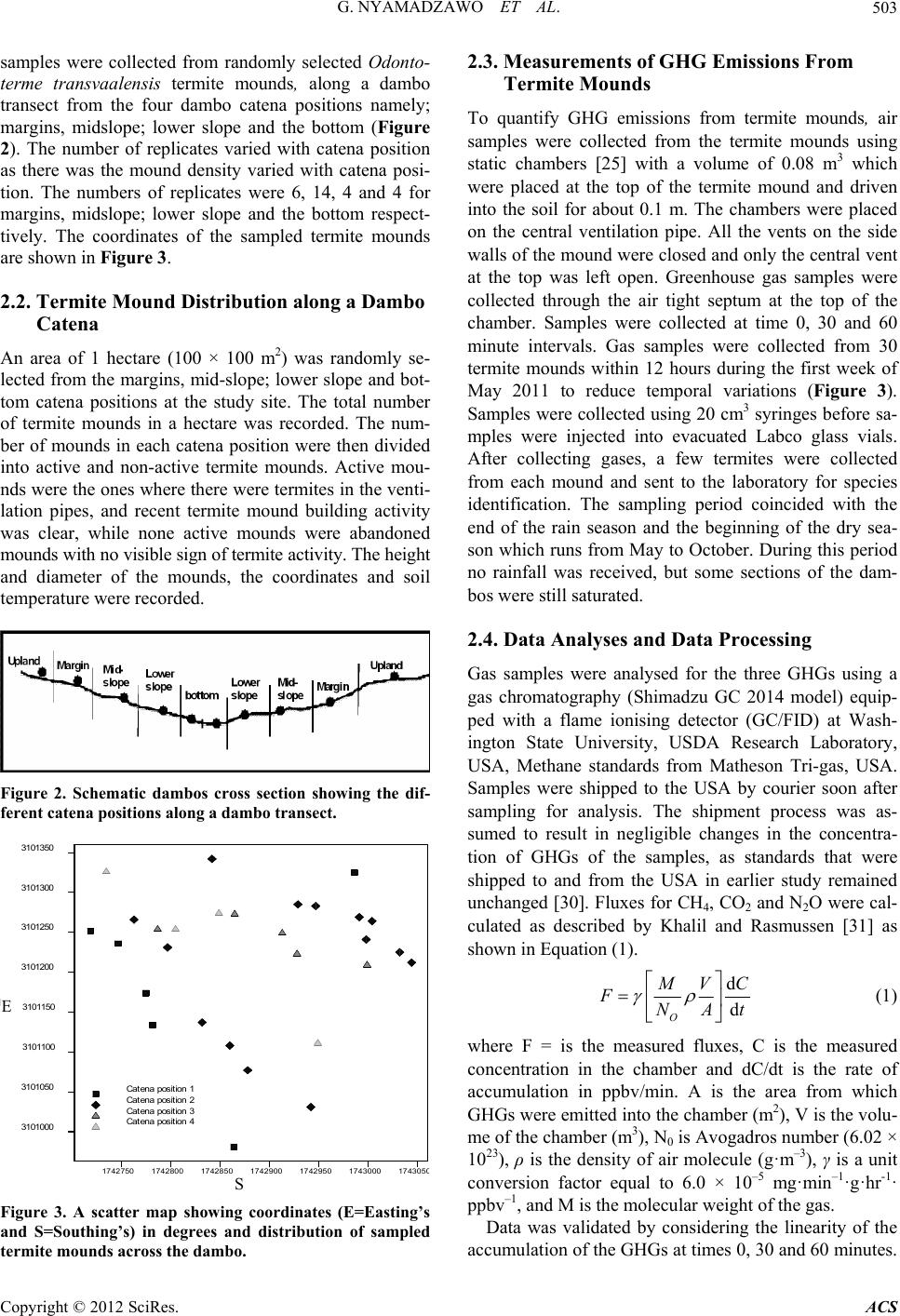 G. NYAMADZAWO ET AL. 503 samples were collected from randomly selected Odonto- terme transvaalensis termite mounds, along a dambo transect from the four dambo catena positions namely; margins, midslope; lower slope and the bottom (Figure 2). The number of replicates varied with catena position as there was the mound density varied with catena posi- tion. The numbers of replicates were 6, 14, 4 and 4 for margins, midslope; lower slope and the bottom respect- tively. The coordinates of the sampled termite mounds are shown in Figure 3. 2.2. Termite Mound Distribution along a Dambo Catena An area of 1 hectare (100 × 100 m2) was randomly se- lected from the margins, mid-slope; lower slope and bot- tom catena positions at the study site. The total number of termite mounds in a hectare was recorded. The num- ber of mounds in each catena position were then divided into active and non-active termite mounds. Active mou- nds were the ones where there were termites in the venti- lation pipes, and recent termite mound building activity was clear, while none active mounds were abandoned mounds with no visible sign of termite activity. The height and diameter of the mounds, the coordinates and soil temperature were recorded. Figure 2. Schematic dambos cross section showing the dif- ferent catena positions along a dambo transect. 3101200 1742800 174305 1743000 3101050 3101150 1742950 3101300 1742900 3101000 3101100 1742750 3101250 3101350 1742850 E Catena position 3 Catena position 4 Catena position 1 Catena position 2 S E Figure 3. A scatter map showing coordinates (E=Easting’s and S=Southing’s) in degrees and distribution of sampled termite mounds across the dambo. 2.3. Measurements of GHG Emissions From Termite Mounds To quantify GHG emissions from termite mounds, air samples were collected from the termite mounds using static chambers [25] with a volume of 0.08 m3 which were placed at the top of the termite mound and driven into the soil for about 0.1 m. The chambers were placed on the central ventilation pipe. All the vents on the side walls of the mound were closed and only the central vent at the top was left open. Greenhouse gas samples were collected through the air tight septum at the top of the chamber. Samples were collected at time 0, 30 and 60 minute intervals. Gas samples were collected from 30 termite mounds within 12 hours during the first week of May 2011 to reduce temporal variations (Figure 3). Samples were collected using 20 cm3 syringes before sa- mples were injected into evacuated Labco glass vials. After collecting gases, a few termites were collected from each mound and sent to the laboratory for species identification. The sampling period coincided with the end of the rain season and the beginning of the dry sea- son which runs from May to October. During this period no rainfall was received, but some sections of the dam- bos were still saturated. 2.4. Data Analyses and Data Processing Gas samples were analysed for the three GHGs using a gas chromatography (Shimadzu GC 2014 model) equip- ped with a flame ionising detector (GC/FID) at Wash- ington State University, USDA Research Laboratory, USA, Methane standards from Matheson Tri-gas, USA. Samples were shipped to the USA by courier soon after sampling for analysis. The shipment process was as- sumed to result in negligible changes in the concentra- tion of GHGs of the samples, as standards that were shipped to and from the USA in earlier study remained unchanged [30]. Fluxes for CH4, CO2 and N2O were cal- culated as described by Khalil and Rasmussen [31] as shown in Equation (1). d d O VC FNAt (1) where F = is the measured fluxes, C is the measured concentration in the chamber and dC/dt is the rate of accumulation in ppbv/min. A is the area from which GHGs were emitted into the chamber (m2), V is the volu- me of the chamber (m3), N0 is Avogadros number (6.02 × 1023), ρ is the density of air molecule (g·m–3), γ is a unit conversion factor equal to 6.0 × 10–5 mg·min–1·g·hr-1· ppbv–1, and M is the molecular weight of the gas. Data was validated by considering the linearity of the accumulation of the GHGs at times 0, 30 and 60 minutes. Copyright © 2012 SciRes. ACS
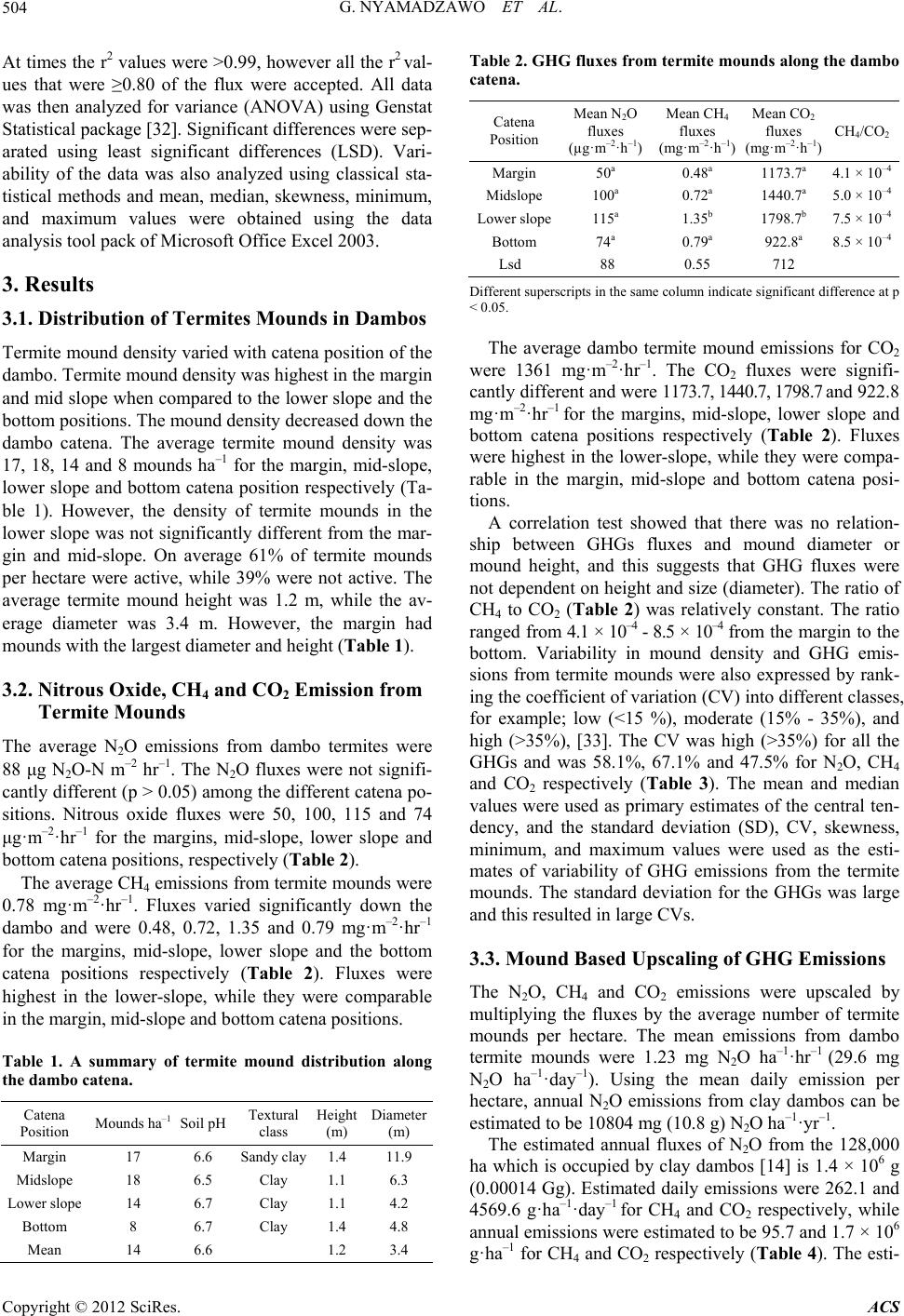 G. NYAMADZAWO ET AL. 504 At times the r2 values were >0.99, however all the r2 val- ues that were ≥0.80 of the flux were accepted. All data was then analyzed for variance (ANOVA) using Genstat Statistical package [32]. Significant differences were sep- arated using least significant differences (LSD). Vari- ability of the data was also analyzed using classical sta- tistical methods and mean, median, skewness, minimum, and maximum values were obtained using the data analysis tool pack of Microsoft Office Excel 2003. 3. Results 3.1. Distribution of Termites Mounds in Dambos Termite mound density varied with catena position of the dambo. Termite mound density was highest in the margin and mid slope when compared to the lower slope and the bottom positions. The mound density decreased down the dambo catena. The average termite mound density was 17, 18, 14 and 8 mounds ha–1 for the margin, mid-slope, lower slope and bottom catena position respectively (Ta- ble 1). However, the density of termite mounds in the lower slope was not significantly different from the mar- gin and mid-slope. On average 61% of termite mounds per hectare were active, while 39% were not active. The average termite mound height was 1.2 m, while the av- erage diameter was 3.4 m. However, the margin had mounds with the largest diameter and height (Table 1). 3.2. Nitrous Oxide, CH4 and CO2 Emission from Termite Mounds The average N2O emissions from dambo termites were 88 μg N2O-N m–2 hr–1. The N2O fluxes were not signifi- cantly different (p > 0.05) among the different catena po- sitions. Nitrous oxide fluxes were 50, 100, 115 and 74 μg·m–2·hr–1 for the margins, mid-slope, lower slope and bottom catena positions, respectively (Table 2). The average CH4 emissions from termite mounds were 0.78 mg·m–2·hr–1. Fluxes varied significantly down the dambo and were 0.48, 0.72, 1.35 and 0.79 mg·m–2·hr–1 for the margins, mid-slope, lower slope and the bottom catena positions respectively (Table 2). Fluxes were highest in the lower-slope, while they were comparable in the margin, mid-slope and bottom catena positions. Table 1. A summary of termite mound distribution along the dambo catena. Catena Position Mounds ha–1 Soil pHTextural class Height (m) Diameter (m) Margin 17 6.6 Sandy clay 1.4 11.9 Midslope 18 6.5 Clay 1.1 6.3 Lower slope 14 6.7 Clay 1.1 4.2 Bottom 8 6.7 Clay 1.4 4.8 Mean 14 6.6 1.2 3.4 Table 2. GHG fluxes from termite mounds along the dambo catena. Catena Position Mean N2O fluxes (µg·m–2·h–1) Mean CH4 fluxes (mg·m–2·h–1) Mean CO2 fluxes (mg·m–2·h–1) CH4/CO2 Margin 50a 0.48a 1173.7a 4.1 × 10–4 Midslope 100a 0.72a 1440.7a 5.0 × 10–4 Lower slope115a 1.35b 1798.7b 7.5 × 10–4 Bottom 74a 0.79a 922.8a 8.5 × 10–4 Lsd 88 0.55 712 Different superscripts in the same column indicate significant difference at p < 0.05. The average dambo termite mound emissions for CO2 were 1361 mg·m–2·hr–1. The CO2 fluxes were signifi- cantly different and were 1173.7, 1440.7, 1798.7 and 922.8 mg· m–2·hr–1 for the margins, mid-slope, lower slope and bottom catena positions respectively (Table 2). Fluxes were highest in the lower-slope, while they were compa- rable in the margin, mid-slope and bottom catena posi- tions. A correlation test showed that there was no relation- ship between GHGs fluxes and mound diameter or mound height, and this suggests that GHG fluxes were not dependent on height and size (diameter). The ratio of CH4 to CO2 (Table 2) was relatively constant. The ratio ranged from 4.1 × 10–4 - 8.5 × 10–4 from the margin to the bottom. Variability in mound density and GHG emis- sions from termite mounds were also expressed by rank- ing the coefficient of variation (CV) into different classes, for example; low (<15 %), moderate (15% - 35%), and high (>35%), [33]. The CV was high (>35%) for all the GHGs and was 58.1%, 67.1% and 47.5% for N2O, CH4 and CO2 respectively (Table 3). The mean and median values were used as primary estimates of the central ten- dency, and the standard deviation (SD), CV, skewness, minimum, and maximum values were used as the esti- mates of variability of GHG emissions from the termite mounds. The standard deviation for the GHGs was large and this resulted in large CVs. 3.3. Mound Based Upscaling of GHG Emissions The N2O, CH4 and CO2 emissions were upscaled by multiplying the fluxes by the average number of termite mounds per hectare. The mean emissions from dambo termite mounds were 1.23 mg N2O ha–1·hr–1 (29.6 mg N2O ha–1·day–1). Using the mean daily emission per hectare, annual N2O emissions from clay dambos can be estimated to be 10804 mg (10.8 g) N2O ha–1·yr–1. The estimated annual fluxes of N2O from the 128,000 ha which is occupied by clay dambos [14] is 1.4 × 106 g (0.00014 Gg). Estimated daily emissions were 262.1 and 4569.6 g·ha–1·day–1 for CH4 and CO2 respectively, while annual emissions were estimated to be 95.7 and 1.7 × 106 g·ha–1 for CH4 and CO2 respectively (Table 4). The esti- Copyright © 2012 SciRes. ACS
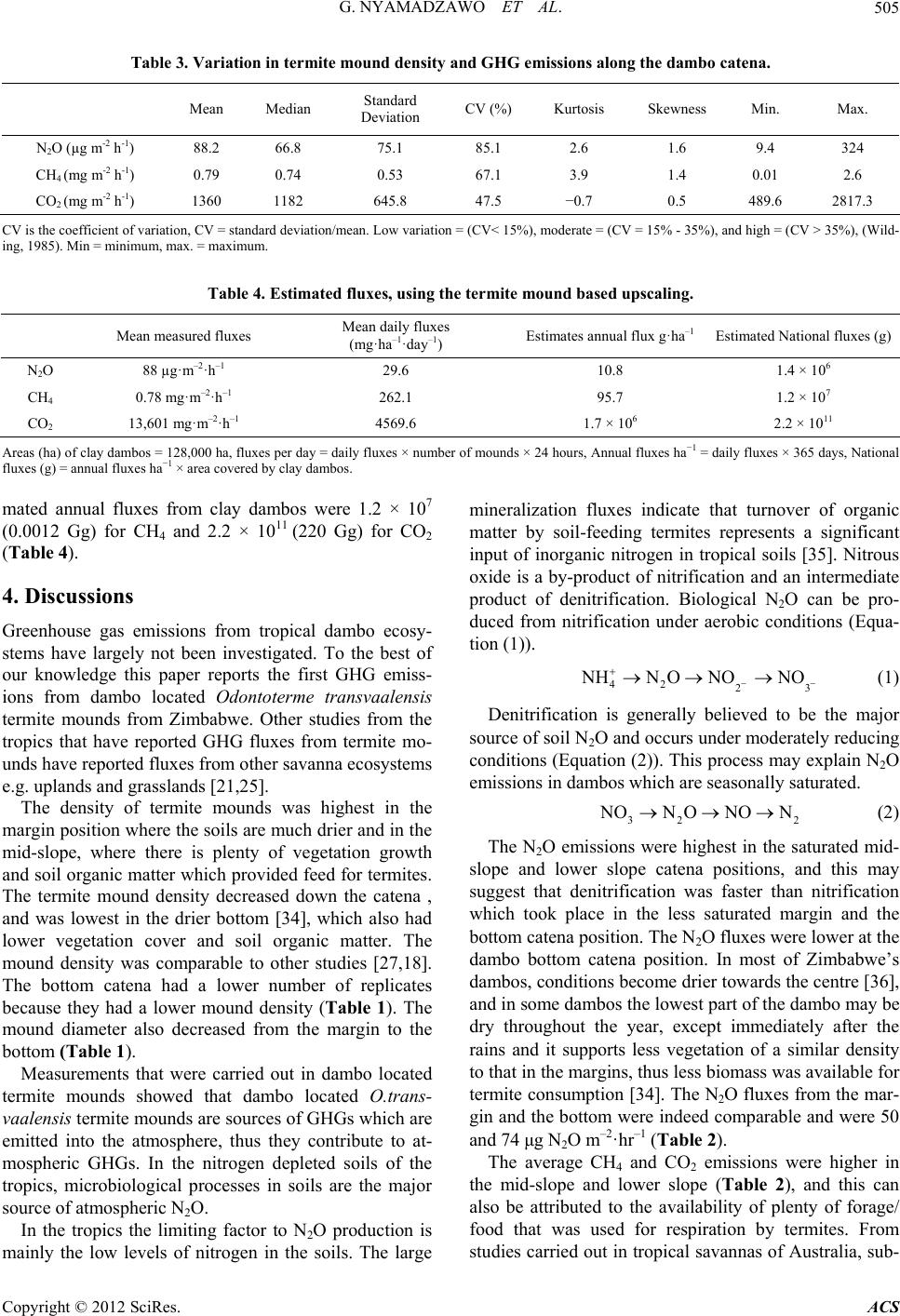 G. NYAMADZAWO ET AL. Copyright © 2012 SciRes. ACS 505 Table 3. Variation in termite mound density and GHG emissions along the dambo catena. Mean Median Standard Deviation CV (%) Kurtosis Skewness Min. Max. N2O (µg m-2 h-1) 88.2 66.8 75.1 85.1 2.6 1.6 9.4 324 CH4 (mg m-2 h-1) 0.79 0.74 0.53 67.1 3.9 1.4 0.01 2.6 CO2 (mg m-2 h-1) 1360 1182 645.8 47.5 −0.7 0.5 489.6 2817.3 CV is the coefficient of variation, CV = standard deviation/mean. Low variation = (CV< 15%), moderate = (CV = 15% - 35%), and high = (CV > 35%), (Wild- ing, 1985). Min = minimum, max. = maximum. Table 4. Estimated fluxes, using the termite mound based upscaling. Mean measured fluxes Mean daily fluxes (mg·ha–1·day–1) Estimates annual flux g·ha–1 Estimated National fluxes (g) N2O 88 µg·m–2·h–1 29.6 10.8 1.4 × 106 CH4 0.78 mg·m–2·h–1 262.1 95.7 1.2 × 107 CO2 13,601 mg·m–2·h–1 4569.6 1.7 × 106 2.2 × 1011 Areas (ha) of clay dambos = 128,000 ha, fluxes per day = daily fluxes × number of mounds × 24 hours, Annual fluxes ha−1 = daily fluxes × 365 days, National fluxes (g) = annual fluxes ha−1 × area covered by clay dambos. mated annual fluxes from clay dambos were 1.2 × 107 (0.0012 Gg) for CH4 and 2.2 × 1011 (220 Gg) for CO2 (Table 4). 4. Discussions Greenhouse gas emissions from tropical dambo ecosy- stems have largely not been investigated. To the best of our knowledge this paper reports the first GHG emiss- ions from dambo located Odontoterme transvaalensis termite mounds from Zimbabwe. Other studies from the tropics that have reported GHG fluxes from termite mo- unds have reported fluxes from other savanna ecosystems e.g. uplands and grasslands [21,25]. The density of termite mounds was highest in the margin position where the soils are much drier and in the mid-slope, where there is plenty of vegetation growth and soil organic matter which provided feed for termites. The termite mound density decreased down the catena , and was lowest in the drier bottom [34], which also had lower vegetation cover and soil organic matter. The mound density was comparable to other studies [27,18]. The bottom catena had a lower number of replicates because they had a lower mound density (Table 1). The mound diameter also decreased from the margin to the bottom (Table 1). Measurements that were carried out in dambo located termite mounds showed that dambo located O.trans- vaalensis termite mounds are sources of GHGs which are emitted into the atmosphere, thus they contribute to at- mospheric GHGs. In the nitrogen depleted soils of the tropics, microbiological processes in soils are the major source of atmospheric N2O. In the tropics the limiting factor to N2O production is mainly the low levels of nitrogen in the soils. The large mineralization fluxes indicate that turnover of organic matter by soil-feeding termites represents a significant input of inorganic nitrogen in tropical soils [35]. Nitrous oxide is a by-product of nitrification and an intermediate product of denitrification. Biological N2O can be pro- duced from nitrification under aerobic conditions (Equa- tion (1)). 42 23 HNONO NO 32 2 (1) Denitrification is generally believed to be the major source of soil N2O and occurs under moderately reducing conditions (Equation (2)). This process may explain N2O emissions in dambos which are seasonally saturated. O NONON (2) The N2O emissions were highest in the saturated mid- slope and lower slope catena positions, and this may suggest that denitrification was faster than nitrification which took place in the less saturated margin and the bottom catena position. The N2O fluxes were lower at the dambo bottom catena position. In most of Zimbabwe’s dambos, conditions become drier towards the centre [36], and in some dambos the lowest part of the dambo may be dry throughout the year, except immediately after the rains and it supports less vegetation of a similar density to that in the margins, thus less biomass was available for termite consumption [34]. The N2O fluxes from the mar- gin and the bottom were indeed comparable and were 50 and 74 μg N2O m–2·hr–1 (Table 2). The average CH4 and CO2 emissions were higher in the mid-slope and lower slope (Table 2), and this can also be attributed to the availability of plenty of forage/ food that was used for respiration by termites. From studies carried out in tropical savannas of Australia, sub-
 G. NYAMADZAWO ET AL. 506 terranean termites graze about 100 kg·ha–1 [37] and [22] estimated emissions of N2O from termites to be 0.0125% of the weight of the carbon consumed assuming a fixed C:N ratio, and of the carbon consumed by termites, 1.23% (average of measured emissions) is emitted in the form of methane while the remainder is emitted as CO2. The mid-slope and lower slope catena positions also had suitable anaerobic conditions that ensured CH4 form- ation as they were saturated for long periods when com- pared to other catena positions [34,38]. The mid-slope and lower slope catena positions also showed decreasing soil particle size (9,13] and increasing proportion of ac- tive clays relative to kaolinite [16]. The nature of the clay affects CH4 emission because some clay types protect organic matter from mineralisation which delays methan- ogenesis, while soils rich in swelling clays (active) are usually more favourable to methanogenesis than sandy soils or soils rich in kaolinite [5]. The mean N2O fluxes from dambo termite mounds reported in this paper are higher than the end of rain sea- son means reported for other termite mounds from Africa. [28] reported dry period fluxes of 6 - 36 μg N2O m–2·hr–1, with mean fluxes of 20 μg N2O m–2·hr–1 at the end of the rainy season from upland termite mounds from tropical savannas of Burkina Faso. Higher fluxes (88 μg N2O m–2·hr–1) from the dambo termite mounds at the end of the rainy season can be a result of plenty of vegetation and soil organic matter which is consumed by the ter- mites in these organic matter rich dambos. However, the N2O emissions from termite mounds reported in this study were comparable to re-calculated fluxes from tem- perate forest soils of 10 - 17 g N2O ha–1·yr–1 [39]. The mean N2O fluxes reported in this study are higher and more than double the fluxes reported from other ecosystems of the savannas in Zimbabwe and the region, showing that termite mounds are a hotspot for N2O. [40] reported N2O fluxes ranging from 0.5 to 35.7 μg N2O m–2·hr –1 in upland soils at the same study site from the dry to the rain season. [41] reported highest N2O fluxes of 42 μg N2O m–2·hr –1 from miombo woodlands, while Werner et al. (2007) reported mean N2O emissions of 42.9 ± 0.7 µg N m−2·hr−1 from tropical rain forest in Kenya. Methane fluxes from O. transvaalensis termite mounds which ranged from 480 - 1350 μg·m–2·hr–1 were higher than fluxes reported from other tropical ecosystems in Zimbabwe. [40] reported CH4 emissions ranging from 1.9 - 102 μg·m–2·hr–1 under different landuse systems in Zimbabwe. However, CH4 emissions from dambo ter- mites mounds (mean = 0.78 and range 0.48 - 1.35 mg· m–2·hr–1) were 12 - 13.5 times higher than the emissions from Odontotermes from South Africa which were lo- cated in savannas with dense grass cover and some un- derbrush and had a range of 0.04 - 0.1 mg/nest/hour [25]. The higher fluxes in dambo termite mounds from this study could be attributed to huge amounts of vegetation and soil organic carbon additions in dambos when com- pared to other savannas that were used in the study by [25]. However, fluxes from termite mounds were lower than emissions reported 9 mg CH4 m –2 hr–1 or 216 mg CH4 m –2 day–1, by [42,43] from seasonally saturated dambos. Mapanda et al. (2010) also reported much lower emis- sions for CO2 for other non-dambo tropical ecosystems (8.4-190.4 mg m–2 hr–1) when compared to a range from 922.9-1798.7 mg m–2 hr–1 from dambo based O. trans- vaalensis termite mounds. The measured CO2 emissions were also lower than the reported values by [25], how- ever the CH4/CO2 ratios were comparable. The higher N2O and CO2 emissions from dambo located termite mounds could be attributed to the presence of abundant plant material, organic matter, higher termite populations and residual soil moisture in dambos such that dambo termites would have abundant plant materials to feed on. The GHG fluxes from dambo termite mounds were in- dependent of mound diameter or mound height and soil temperature. The ratio of CH4 to CO2 were also inde- pendent of catena position, mound diameter or mound height, soil temperature and a similar observation was made by [25]. Greenhouse gas emissions from termite mounds showed higher standard deviation and a corresponding higher CV (Table 3). The high CV for GHGs may be due to intrinsic (natural variations) or extrinsic (imposed) sources of variability such as management. Intrinsic vari- ability is natural variation in soils [44] and extrinsic vari- ability is caused by factors imposed on a site [45]. Intrin- sic factors such as variations in degree of saturation, type of vegetation, clay content and clay mineralogy could also have contributed to the variations in GHG emissions across the dambos. With an estimated emission of 10.8, 95.6 and 1.7 × 106 g·ha–1 annually for N2O, CH4 and CO2 respectively (Ta- ble 4), it is apparent that dambo O. transvaalensis termite mounds are an important source of GHGs. Greenhouse gas emissions from dambo termite mounds reported in this study were comparable to other ecosystem e.g. tem- perate N2O emissions [39] and higher than CH4 emis- sions from O. transvaalensis termite mounds from dry tropical soils [25]. Although some studies have argued that the actual termite contribution to gas fluxes re- mained small in the global context [46,47], the contribu- tions of dambo located O. transvaalensis termites to wards total N2O, CO2 and CH4 emissions from dambos and from the seasonally dry ecosystems of Africa cannot be ignored. Thus, GHG emissions from dambo located termite mounds should be accounted for when making estimates of N2O emissions from the tropics. Copyright © 2012 SciRes. ACS
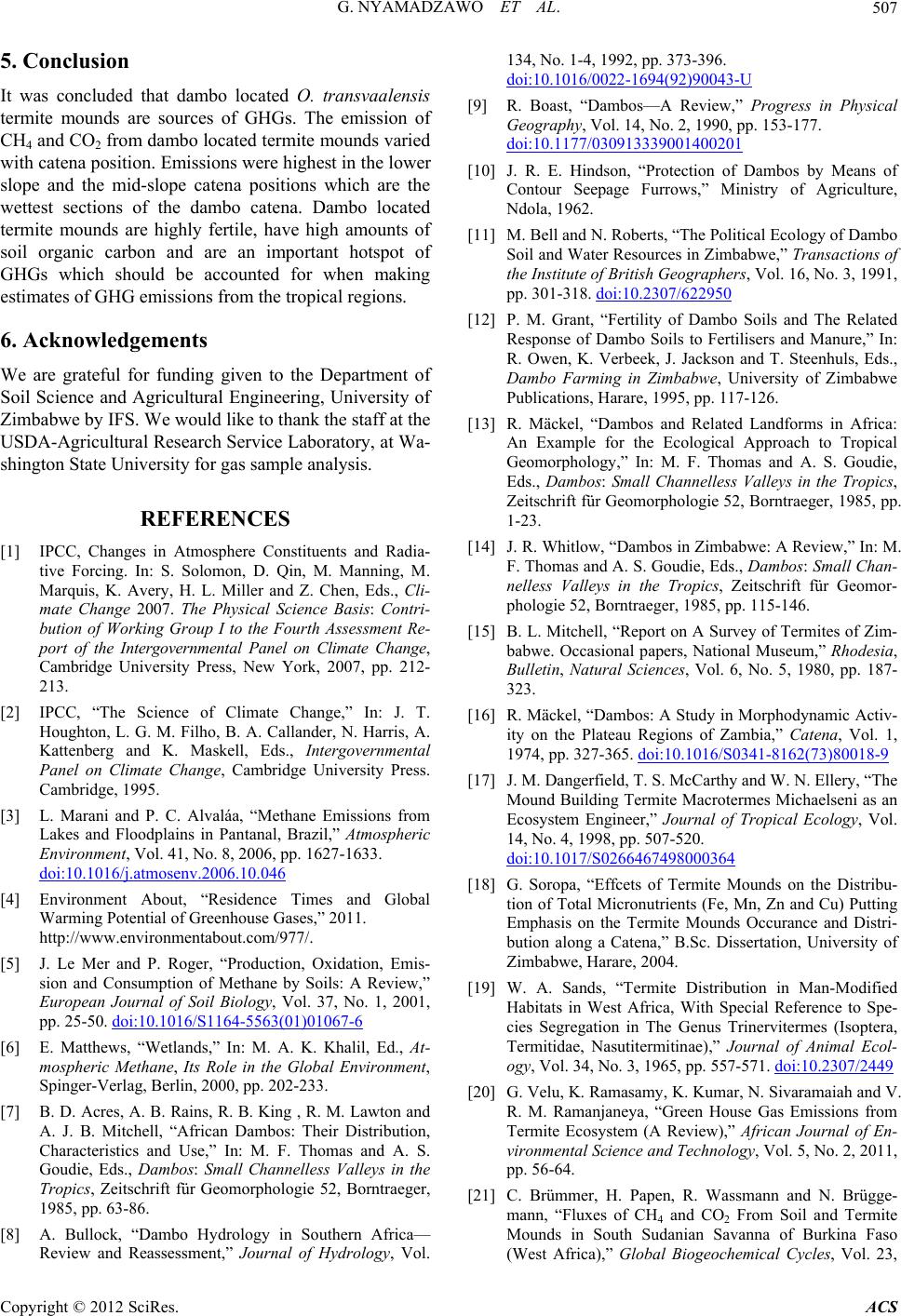 G. NYAMADZAWO ET AL. 507 5. Conclusion It was concluded that dambo located O. transvaalensis termite mounds are sources of GHGs. The emission of CH4 and CO2 from dambo located termite mounds varied with catena position. Emissions were highest in the lower slope and the mid-slope catena positions which are the wettest sections of the dambo catena. Dambo located termite mounds are highly fertile, have high amounts of soil organic carbon and are an important hotspot of GHGs which should be accounted for when making estimates of GHG emissions from the tropical regions. 6. Acknowledgements We are grateful for funding given to the Department of Soil Science and Agricultural Engineering, University of Zimbabwe by IFS. We would like to thank the staff at the USDA-Agricultural Research Service Laboratory, at Wa- shington State University for gas sample analysis. REFERENCES [1] IPCC, Changes in Atmosphere Constituents and Radia- tive Forcing. In: S. Solomon, D. Qin, M. Manning, M. Marquis, K. Avery, H. L. Miller and Z. Chen, Eds., Cli- mate Change 2007. The Physical Science Basis: Contri- bution of Working Group I to the Fourth Assessment Re- port of the Intergovernmental Panel on Climate Change, Cambridge University Press, New York, 2007, pp. 212- 213. [2] IPCC, “The Science of Climate Change,” In: J. T. Houghton, L. G. M. Filho, B. A. Callander, N. Harris, A. Kattenberg and K. Maskell, Eds., Intergovernmental Panel on Climate Change, Cambridge University Press. Cambridge, 1995. [3] L. Marani and P. C. Alvaláa, “Methane Emissions from Lakes and Floodplains in Pantanal, Brazil,” Atmospheric Environment, Vol. 41, No. 8, 2006, pp. 1627-1633. doi:10.1016/j.atmosenv.2006.10.046 [4] Environment About, “Residence Times and Global Warming Potential of Greenhouse Gases,” 2011. http://www.environmentabout.com/977/. [5] J. Le Mer and P. Roger, “Production, Oxidation, Emis- sion and Consumption of Methane by Soils: A Review,” European Journal of Soil Biology, Vol. 37, No. 1, 2001, pp. 25-50. doi:10.1016/S1164-5563(01)01067-6 [6] E. Matthews, “Wetlands,” In: M. A. K. Khalil, Ed., At- mospheric Methane, Its Role in the Global Environment, Spinger-Verlag, Berlin, 2000, pp. 202-233. [7] B. D. Acres, A. B. Rains, R. B. King , R. M. Lawton and A. J. B. Mitchell, “African Dambos: Their Distribution, Characteristics and Use,” In: M. F. Thomas and A. S. Goudie, Eds., Dambos: Small Channelless Valleys in the Tropics, Zeitschrift für Geomorphologie 52, Borntraeger, 1985, pp. 63-86. [8] A. Bullock, “Dambo Hydrology in Southern Africa— Review and Reassessment,” Journal of Hydrology, Vol. 134, No. 1-4, 1992, pp. 373-396. doi:10.1016/0022-1694(92)90043-U [9] R. Boast, “Dambos—A Review,” Progress in Physical Geography, Vol. 14, No. 2, 1990, pp. 153-177. doi:10.1177/030913339001400201 [10] J. R. E. Hindson, “Protection of Dambos by Means of Contour Seepage Furrows,” Ministry of Agriculture, Ndola, 1962. [11] M. Bell and N. Roberts, “The Political Ecology of Dambo Soil and Water Resources in Zimbabwe,” Transactions of the Institute of British Geographers, Vol. 16, No. 3, 1991, pp. 301-318. doi:10.2307/622950 [12] P. M. Grant, “Fertility of Dambo Soils and The Related Response of Dambo Soils to Fertilisers and Manure,” In: R. Owen, K. Verbeek, J. Jackson and T. Steenhuls, Eds., Dambo Farming in Zimbabwe, University of Zimbabwe Publications, Harare, 1995, pp. 117-126. [13] R. Mäckel, “Dambos and Related Landforms in Africa: An Example for the Ecological Approach to Tropical Geomorphology,” In: M. F. Thomas and A. S. Goudie, Eds., Dambos: Small Channelless Valleys in the Tropics, Zeitschrift für Geomorphologie 52, Borntraeger, 1985, pp. 1-23. [14] J. R. Whitlow, “Dambos in Zimbabwe: A Review,” In: M. F. Thomas and A. S. Goudie, Eds., Dambos: Small Chan- nelless Valleys in the Tropics, Zeitschrift für Geomor- phologie 52, Borntraeger, 1985, pp. 115-146. [15] B. L. Mitchell, “Report on A Survey of Termites of Zim- babwe. Occasional papers, National Museum,” Rhodesia, Bulletin, Natural Sciences, Vol. 6, No. 5, 1980, pp. 187- 323. [16] R. Mäckel, “Dambos: A Study in Morphodynamic Activ- ity on the Plateau Regions of Zambia,” Catena, Vol. 1, 1974, pp. 327-365. doi:10.1016/S0341-8162(73)80018-9 [17] J. M. Dangerfield, T. S. McCarthy and W. N. Ellery, “The Mound Building Termite Macrotermes Michaelseni as an Ecosystem Engineer,” Journal of Tropical Ecology, Vol. 14, No. 4, 1998, pp. 507-520. doi:10.1017/S0266467498000364 [18] G. Soropa, “Effcets of Termite Mounds on the Distribu- tion of Total Micronutrients (Fe, Mn, Zn and Cu) Putting Emphasis on the Termite Mounds Occurance and Distri- bution along a Catena,” B.Sc. Dissertation, University of Zimbabwe, Harare, 2004. [19] W. A. Sands, “Termite Distribution in Man-Modified Habitats in West Africa, With Special Reference to Spe- cies Segregation in The Genus Trinervitermes (Isoptera, Termitidae, Nasutitermitinae),” Journal of Animal Ecol- ogy, Vol. 34, No. 3, 1965, pp. 557-571. doi:10.2307/2449 [20] G. Velu, K. Ramasamy, K. Kumar, N. Sivaramaiah and V. R. M. Ramanjaneya, “Green House Gas Emissions from Termite Ecosystem (A Review),” African Journal of En- vironmental Science and Technology, Vol. 5, No. 2, 2011, pp. 56-64. [21] C. Brümmer, H. Papen, R. Wassmann and N. Brügge- mann, “Fluxes of CH4 and CO2 From Soil and Termite Mounds in South Sudanian Savanna of Burkina Faso (West Africa),” Global Biogeochemical Cycles, Vol. 23, Copyright © 2012 SciRes. ACS
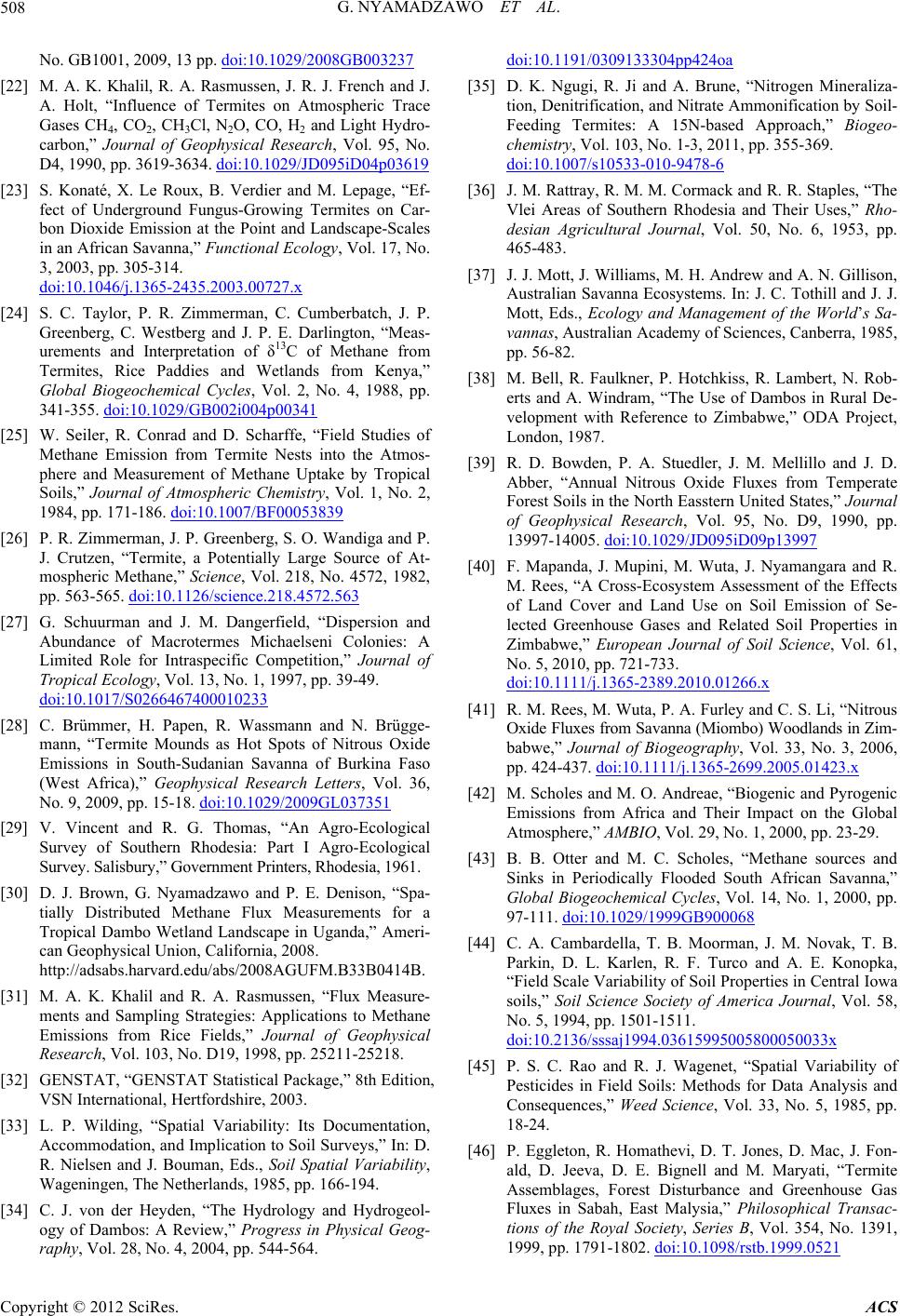 G. NYAMADZAWO ET AL. 508 No. GB1001, 2009, 13 pp. doi:10.1029/2008GB003237 [22] M. A. K. Khalil, R. A. Rasmussen, J. R. J. French and J. A. Holt, “Influence of Termites on Atmospheric Trace Gases CH4, CO2, CH3Cl, N2O, CO, H2 and Light Hydro- carbon,” Journal of Geophysical Research, Vol. 95, No. D4, 1990, pp. 3619-3634. doi:10.1029/JD095iD04p03619 [23] S. Konaté, X. Le Roux, B. Verdier and M. Lepage, “Ef- fect of Underground Fungus-Growing Termites on Car- bon Dioxide Emission at the Point and Landscape-Scales in an African Savanna,” Functional Ecology, Vol. 17, No. 3, 2003, pp. 305-314. doi:10.1046/j.1365-2435.2003.00727.x [24] S. C. Taylor, P. R. Zimmerman, C. Cumberbatch, J. P. Greenberg, C. Westberg and J. P. E. Darlington, “Meas- urements and Interpretation of δ13C of Methane from Termites, Rice Paddies and Wetlands from Kenya,” Global Biogeochemical Cycles, Vol. 2, No. 4, 1988, pp. 341-355. doi:10.1029/GB002i004p00341 [25] W. Seiler, R. Conrad and D. Scharffe, “Field Studies of Methane Emission from Termite Nests into the Atmos- phere and Measurement of Methane Uptake by Tropical Soils,” Journal of Atmospheric Chemistry, Vol. 1, No. 2, 1984, pp. 171-186. doi:10.1007/BF00053839 [26] P. R. Zimmerman, J. P. Greenberg, S. O. Wandiga and P. J. Crutzen, “Termite, a Potentially Large Source of At- mospheric Methane,” Science, Vol. 218, No. 4572, 1982, pp. 563-565. doi:10.1126/science.218.4572.563 [27] G. Schuurman and J. M. Dangerfield, “Dispersion and Abundance of Macrotermes Michaelseni Colonies: A Limited Role for Intraspecific Competition,” Journal of Tropical Ecology, Vol. 13, No. 1, 1997, pp. 39-49. doi:10.1017/S0266467400010233 [28] C. Brümmer, H. Papen, R. Wassmann and N. Brügge- mann, “Termite Mounds as Hot Spots of Nitrous Oxide Emissions in South-Sudanian Savanna of Burkina Faso (West Africa),” Geophysical Research Letters, Vol. 36, No. 9, 2009, pp. 15-18. doi:10.1029/2009GL037351 [29] V. Vincent and R. G. Thomas, “An Agro-Ecological Survey of Southern Rhodesia: Part I Agro-Ecological Survey. Salisbury,” Government Printers, Rhodesia, 1961. [30] D. J. Brown, G. Nyamadzawo and P. E. Denison, “Spa- tially Distributed Methane Flux Measurements for a Tropical Dambo Wetland Landscape in Uganda,” Ameri- can Geophysical Union, California, 2008. http://adsabs.harvard.edu/abs/2008AGUFM.B33B0414B. [31] M. A. K. Khalil and R. A. Rasmussen, “Flux Measure- ments and Sampling Strategies: Applications to Methane Emissions from Rice Fields,” Journal of Geophysical Research, Vol. 103, No. D19, 1998, pp. 25211-25218. [32] GENSTAT, “GENSTAT Statistical Package,” 8th Edition, VSN International, Hertfordshire, 2003. [33] L. P. Wilding, “Spatial Variability: Its Documentation, Accommodation, and Implication to Soil Surveys,” In: D. R. Nielsen and J. Bouman, Eds., Soil Spatial Variability, Wageningen, The Netherlands, 1985, pp. 166-194. [34] C. J. von der Heyden, “The Hydrology and Hydrogeol- ogy of Dambos: A Review,” Progress in Physical Geog- raphy, Vol. 28, No. 4, 2004, pp. 544-564. doi:10.1191/0309133304pp424oa [35] D. K. Ngugi, R. Ji and A. Brune, “Nitrogen Mineraliza- tion, Denitrification, and Nitrate Ammonification by Soil- Feeding Termites: A 15N-based Approach,” Biogeo- chemistry, Vol. 103, No. 1-3, 2011, pp. 355-369. doi:10.1007/s10533-010-9478-6 [36] J. M. Rattray, R. M. M. Cormack and R. R. Staples, “The Vlei Areas of Southern Rhodesia and Their Uses,” Rho- desian Agricultural Journal, Vol. 50, No. 6, 1953, pp. 465-483. [37] J. J. Mott, J. Williams, M. H. Andrew and A. N. Gillison, Australian Savanna Ecosystems. In: J. C. Tothill and J. J. Mott, Eds., Ecology and Management of the World’s Sa- vannas, Australian Academy of Sciences, Canberra, 1985, pp. 56-82. [38] M. Bell, R. Faulkner, P. Hotchkiss, R. Lambert, N. Rob- erts and A. Windram, “The Use of Dambos in Rural De- velopment with Reference to Zimbabwe,” ODA Project, London, 1987. [39] R. D. Bowden, P. A. Stuedler, J. M. Mellillo and J. D. Abber, “Annual Nitrous Oxide Fluxes from Temperate Forest Soils in the North Easstern United States,” Journal of Geophysical Research, Vol. 95, No. D9, 1990, pp. 13997-14005. doi:10.1029/JD095iD09p13997 [40] F. Mapanda, J. Mupini, M. Wuta, J. Nyamangara and R. M. Rees, “A Cross-Ecosystem Assessment of the Effects of Land Cover and Land Use on Soil Emission of Se- lected Greenhouse Gases and Related Soil Properties in Zimbabwe,” European Journal of Soil Science, Vol. 61, No. 5, 2010, pp. 721-733. doi:10.1111/j.1365-2389.2010.01266.x [41] R. M. Rees, M. Wuta, P. A. Furley and C. S. Li, “Nitrous Oxide Fluxes from Savanna (Miombo) Woodlands in Zim- babwe,” Journal of Biogeography, Vol. 33, No. 3, 2006, pp. 424-437. doi:10.1111/j.1365-2699.2005.01423.x [42] M. Scholes and M. O. Andreae, “Biogenic and Pyrogenic Emissions from Africa and Their Impact on the Global Atmosphere,” AMBIO, Vol. 29, No. 1, 2000, pp. 23-29. [43] B. B. Otter and M. C. Scholes, “Methane sources and Sinks in Periodically Flooded South African Savanna,” Global Biogeochemical Cycles, Vol. 14, No. 1, 2000, pp. 97-111. doi:10.1029/1999GB900068 [44] C. A. Cambardella, T. B. Moorman, J. M. Novak, T. B. Parkin, D. L. Karlen, R. F. Turco and A. E. Konopka, “Field Scale Variability of Soil Properties in Central Iowa soils,” Soil Science Society of America Journal, Vol. 58, No. 5, 1994, pp. 1501-1511. doi:10.2136/sssaj1994.03615995005800050033x [45] P. S. C. Rao and R. J. Wagenet, “Spatial Variability of Pesticides in Field Soils: Methods for Data Analysis and Consequences,” Weed Science, Vol. 33, No. 5, 1985, pp. 18-24. [46] P. Eggleton, R. Homathevi, D. T. Jones, D. Mac, J. Fon- ald, D. Jeeva, D. E. Bignell and M. Maryati, “Termite Assemblages, Forest Disturbance and Greenhouse Gas Fluxes in Sabah, East Malysia,” Philosophical Transac- tions of the Royal Society, Series B, Vol. 354, No. 1391, 1999, pp. 1791-1802. doi:10.1098/rstb.1999.0521 Copyright © 2012 SciRes. ACS
 G. NYAMADZAWO ET AL. Copyright © 2012 SciRes. ACS 509 [47] A. Sugimoto, D. E. Bignell and J. A. MacDonald, “Global Impact of Termites on the Carbon Cycle and Atmospheric Trace Gases,” In: T. Abe, D. E. Bignell and M. Higashi, Eds., Termites: Evolution, Sociality, Symbiosis, Ecology, Kluwer Academic Publishers, Dordrecht, 2000, pp. 409-435.
|