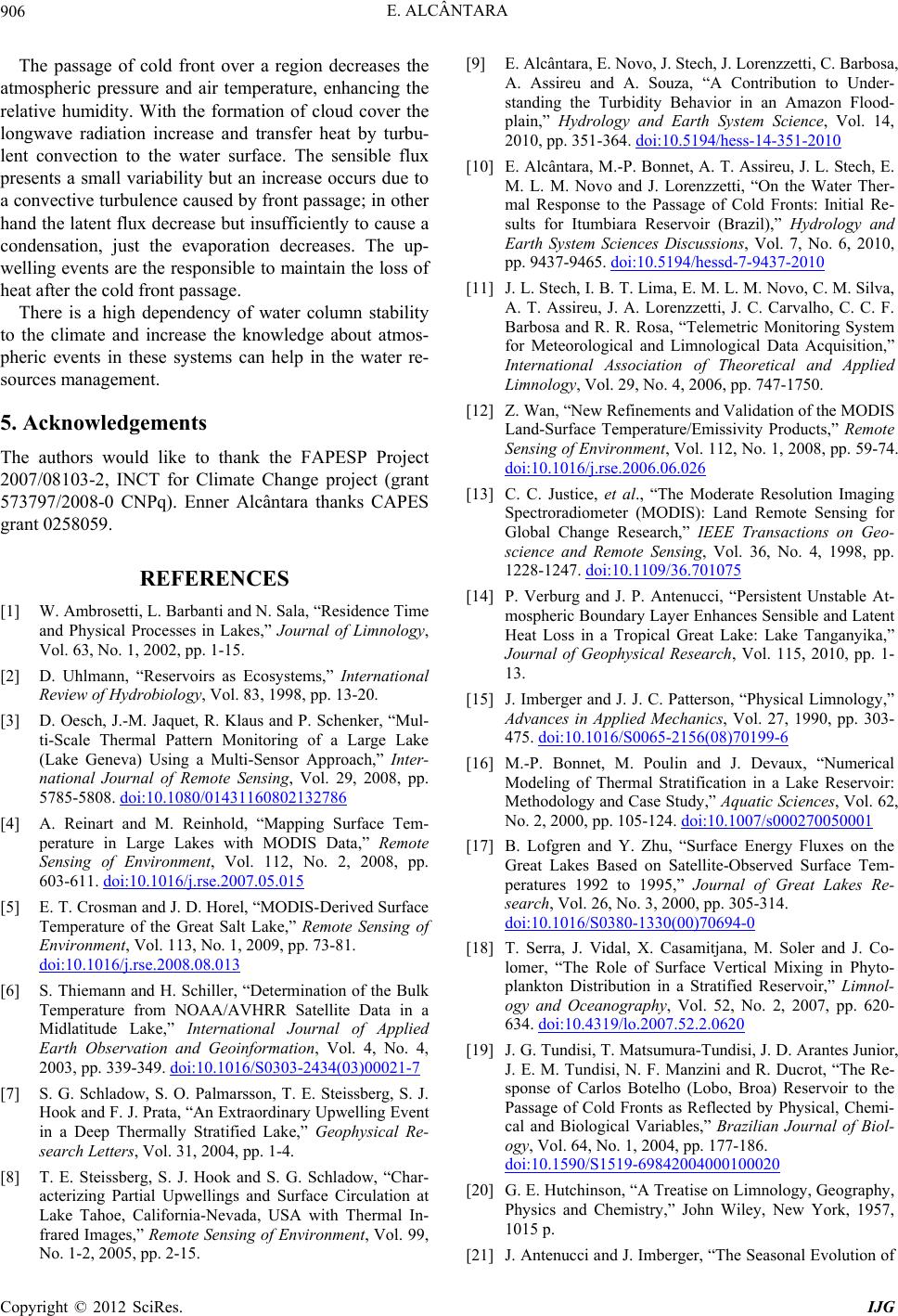
E. ALCÂNTARA
906
The passage of cold front over a region decreases the
atmospheric pressure and air temperature, enhancing the
relative humidity. With the formation of cloud cover the
longwave radiation increase and transfer heat by turbu-
lent convection to the water surface. The sensible flux
presents a small variability but an increase occurs due to
a convective turbulence caused by front passage; in other
hand the latent flux decrease but insufficiently to cause a
condensation, just the evaporation decreases. The up-
welling events are the responsible to maintain the loss of
heat after the cold front passage.
There is a high dependency of water column stability
to the climate and increase the knowledge about atmos-
pheric events in these systems can help in the water re-
sources management.
5. Acknowledgements
The authors would like to thank the FAPESP Project
2007/08103-2, INCT for Climate Change project (grant
573797/2008-0 CNPq). Enner Alcântara thanks CAPES
grant 0258059.
REFERENCES
[1] W. Ambrosetti, L. Barbanti and N. Sala, “Residence Time
and Physical Processes in Lakes,” Journal of Limnology,
Vol. 63, No. 1, 2002, pp. 1-15.
[2] D. Uhlmann, “Reservoirs as Ecosystems,” International
Review of Hydrobiology, Vol. 83, 1998, pp. 13-20.
[3] D. Oesch, J.-M. Jaquet, R. Klaus and P. Schenker, “Mul-
ti-Scale Thermal Pattern Monitoring of a Large Lake
(Lake Geneva) Using a Multi-Sensor Approach,” Inter-
national Journal of Remote Sensing, Vol. 29, 2008, pp.
5785-5808. doi:10.1080/01431160802132786
[4] A. Reinart and M. Reinhold, “Mapping Surface Tem-
perature in Large Lakes with MODIS Data,” Remote
Sensing of Environment, Vol. 112, No. 2, 2008, pp.
603-611. doi:10.1016/j.rse.2007.05.015
[5] E. T. Crosman and J. D. Horel, “MODIS-Derived Surface
Temperature of the Great Salt Lake,” Remote Sensing of
Environment, Vol. 113, No. 1, 2009, pp. 73-81.
doi:10.1016/j.rse.2008.08.013
[6] S. Thiemann and H. Schiller, “Determination of the Bulk
Temperature from NOAA/AVHRR Satellite Data in a
Midlatitude Lake,” International Journal of Applied
Earth Observation and Geoinformation, Vol. 4, No. 4,
2003, pp. 339-349. doi:10.1016/S0303-2434(03)00021-7
[7] S. G. Schladow, S. O. Palmarsson, T. E. Steissberg, S. J.
Hook and F. J. Prata, “An Extraordinary Upwelling Event
in a Deep Thermally Stratified Lake,” Geophysical Re-
search Letters, Vol. 31, 2004, pp. 1-4.
[8] T. E. Steissberg, S. J. Hook and S. G. Schladow, “Char-
acterizing Partial Upwellings and Surface Circulation at
Lake Tahoe, California-Nevada, USA with Thermal In-
frared Images,” Remote Sensing of Environment, Vol. 99,
No. 1-2, 2005, pp. 2-15.
[9] E. Alcântara, E. Novo, J. Stech, J. Lorenzzetti, C. Barbosa,
A. Assireu and A. Souza, “A Contribution to Under-
standing the Turbidity Behavior in an Amazon Flood-
plain,” Hydrology and Earth System Science, Vol. 14,
2010, pp. 351-364. doi:10.5194/hess-14-351-2010
[10] E. Alcântara, M.-P. Bonnet, A. T. Assireu, J. L. Stech, E.
M. L. M. Novo and J. Lorenzzetti, “On the Water Ther-
mal Response to the Passage of Cold Fronts: Initial Re-
sults for Itumbiara Reservoir (Brazil),” Hydrology and
Earth System Sciences Discussions, Vol. 7, No. 6, 2010,
pp. 9437-9465. doi:10.5194/hessd-7-9437-2010
[11] J. L. Stech, I. B. T. Lima, E. M. L. M. Novo, C. M. Silva,
A. T. Assireu, J. A. Lorenzzetti, J. C. Carvalho, C. C. F.
Barbosa and R. R. Rosa, “Telemetric Monitoring System
for Meteorological and Limnological Data Acquisition,”
International Association of Theoretical and Applied
Limnology, Vol. 29, No. 4, 2006, pp. 747-1750.
[12] Z. Wan, “New Refi nements and Validation of the MODIS
Land-Surface Temperature/Emissivity Products,” Remote
Sensing of Environment, Vol. 112, No. 1, 2008, pp. 59-74.
doi:10.1016/j.rse.2006.06.026
[13] C. C. Justice, et al., “The Moderate Resolution Imaging
Spectroradiometer (MODIS): Land Remote Sensing for
Global Change Research,” IEEE Transactions on Geo-
science and Remote Sensing, Vol. 36, No. 4, 1998, pp.
1228-1247. doi:10.1109/36.701075
[14] P. Verburg and J. P. Antenucci, “Persistent Unstable At-
mospheric Boundary Layer Enhances Sensible and Latent
Heat Loss in a Tropical Great Lake: Lake Tanganyika,”
Journal of Geophysical Research, Vol. 115, 2010, pp. 1-
13.
[15] J. Imberger and J. J. C. Patterson, “Physical Limnology,”
Advances in Applied Mechanics, Vol. 27, 1990, pp. 303-
475. doi:10.1016/S0065-2156(08)70199-6
[16] M.-P. Bonnet, M. Poulin and J. Devaux, “Numerical
Modeling of Thermal Stratification in a Lake Reservoir:
Methodology and Case Study,” Aquatic Sciences, Vol. 62,
No. 2, 2000, pp. 105-124. doi:10.1007/s000270050001
[17] B. Lofgren and Y. Zhu, “Surface Energy Fluxes on the
Great Lakes Based on Satellite-Observed Surface Tem-
peratures 1992 to 1995,” Journal of Great Lakes Re-
search, Vol. 26, No. 3, 2000, pp. 305-314.
doi:10.1016/S0380-1330(00)70694-0
[18] T. Serra, J. Vidal, X. Casamitjana, M. Soler and J. Co-
lomer, “The Role of Surface Vertical Mixing in Phyto-
plankton Distribution in a Stratified Reservoir,” Limnol-
ogy and Oceanography, Vol. 52, No. 2, 2007, pp. 620-
634. doi:10.4319/lo.2007.52.2.0620
[19] J. G. Tundisi, T. Matsumura-Tundisi, J. D. Arantes Junior,
J. E. M. Tundisi, N. F. Manzini and R. Ducrot, “The Re-
sponse of Carlos Botelho (Lobo, Broa) Reservoir to the
Passage of Cold Fronts as Reflected by Physical, Chemi-
cal and Biological Variables,” Brazilian Journal of Biol-
ogy, Vol. 64, No. 1, 2004, pp. 177-186.
doi:10.1590/S1519-69842004000100020
[20] G. E. Hutchinson, “A Treatise on Limnology, Geography,
Physics and Chemistry,” John Wiley, New York, 1957,
1015 p.
[21] J. Antenucci and J. Imberger, “The Seasonal Evolution of
Copyright © 2012 SciRes. IJG