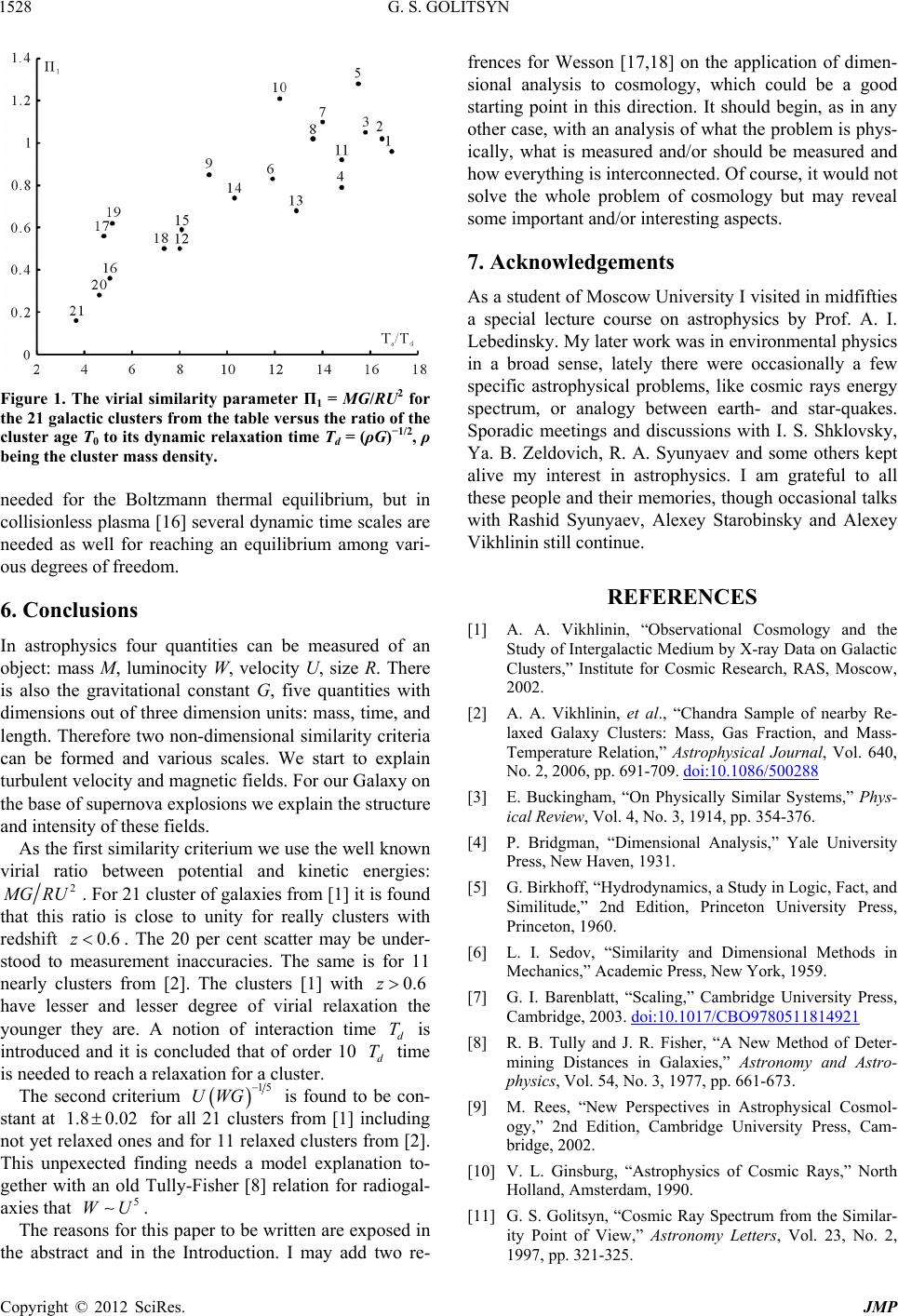
G. S. GOLITSYN
1528
Figure 1. The virial similarity parameter П1 = MG/RU2 for
the 21 galactic clusters from the table versus the ratio of the
cluster age T0 to its dynamic relaxation time Td = (ρG)−1/2, ρ
being the cluster mass density.
needed for the Boltzmann thermal equilibrium, but in
collisionless plasma [16] several dynamic time scales are
needed as well for reaching an equilibrium among vari-
ous degrees of freedom.
6. Conclusions
In astrophysics four quantities can be measured of an
object: mass M, luminocity W, velocity U, size R. There
is also the gravitational constant G, five quantities with
dimensions out of three dimension units: mass, time, and
length. Therefore two non-dimensional similarity criteria
can be formed and various scales. We start to explain
turbulent velocity and magnetic fields. For our Galaxy on
the base of supernova explosions we explain the structure
and intensity of these fields.
As the first similarity criterium we use the well known
virial ratio between potential and kinetic energies:
2
GRU
0.6z
z
T
d
T
. For 21 cluster of galaxies from [1] it is found
that this ratio is close to unity for really clusters with
redshift . The 20 per cent scatter may be under-
stood to measurement inaccuracies. The same is for 11
nearly clusters from [2]. The clusters [1] with
have lesser and lesser degree of virial relaxation the
younger they are. A notion of interaction time d is
introduced and it is concluded that of order 10 time
is needed to reach a relaxation for a cluster.
0.6
The second criterium 15
UWG
1.8
is found to be con-
stant at for all 21 clusters from [1] including
not yet relaxed ones and for 11 relaxed clusters from [2].
This unpexected finding needs a model explanation to-
gether with an old Tully-Fisher [8] relation for radiogal-
axies that .
0.02
5
WU
The reasons for this paper to be written are exposed in
the abstract and in the Introduction. I may add two re-
frences for Wesson [17,18] on the application of dimen-
sional analysis to cosmology, which could be a good
starting point in this direction. It should begin, as in any
other case, with an analysis of what the problem is phys-
ically, what is measured and/or should be measured and
how everything is interconnected. Of course, it would not
solve the whole problem of cosmology but may reveal
some important and/or interesting aspects.
7. Acknowledgements
As a student of Moscow University I visited in midfifties
a special lecture course on astrophysics by Prof. A. I.
Lebedinsky. My later work was in environmental physics
in a broad sense, lately there were occasionally a few
specific astrophysical problems, like cosmic rays energy
spectrum, or analogy between earth- and star-quakes.
Sporadic meetings and discussions with I. S. Shklovsky,
Ya. B. Zeldovich, R. A. Syunyaev and some others kept
alive my interest in astrophysics. I am grateful to all
these people and their memories, though occasional talks
with Rashid Syunyaev, Alexey Starobinsky and Alexey
Vikhlinin still continue.
REFERENCES
[1] A. A. Vikhlinin, “Observational Cosmology and the
Study of Intergalactic Medium by X-ray Data on Galactic
Clusters,” Institute for Cosmic Research, RAS, Moscow,
2002.
[2] A. A. Vikhlinin, et al., “Chandra Sample of nearby Re-
laxed Galaxy Clusters: Mass, Gas Fraction, and Mass-
Temperature Relation,” Astrophysical Journal, Vol. 640,
No. 2, 2006, pp. 691-709. doi:10.1086/500288
[3] E. Buckingham, “On Physically Similar Systems,” Phys-
ical Review, Vol. 4, No. 3, 1914, pp. 354-376.
[4] P. Bridgman, “Dimensional Analysis,” Yale University
Press, New Haven, 1931.
[5] G. Birkhoff, “Hydrodynamics, a Study in Logic, Fact, and
Similitude,” 2nd Edition, Princeton University Press,
Princeton, 1960.
[6] L. I. Sedov, “Similarity and Dimensional Methods in
Mechanics,” Academic Press, New York, 1959.
[7] G. I. Barenblatt, “Scaling,” Cambridge University Press,
Cambridge, 2003. doi:10.1017/CBO9780511814921
[8] R. B. Tully and J. R. Fisher, “A New Method of Deter-
mining Distances in Galaxies,” Astronomy and Astro-
physics, Vol. 54, No. 3, 1977, pp. 661-673.
[9] M. Rees, “New Perspectives in Astrophysical Cosmol-
ogy,” 2nd Edition, Cambridge University Press, Cam-
bridge, 2002.
[10] V. L. Ginsburg, “Astrophysics of Cosmic Rays,” North
Holland, Amsterdam, 1990.
[11] G. S. Golitsyn, “Cosmic Ray Spectrum from the Similar-
ity Point of View,” Astronomy Letters, Vol. 23, No. 2,
1997, pp. 321-325.
Copyright © 2012 SciRes. JMP