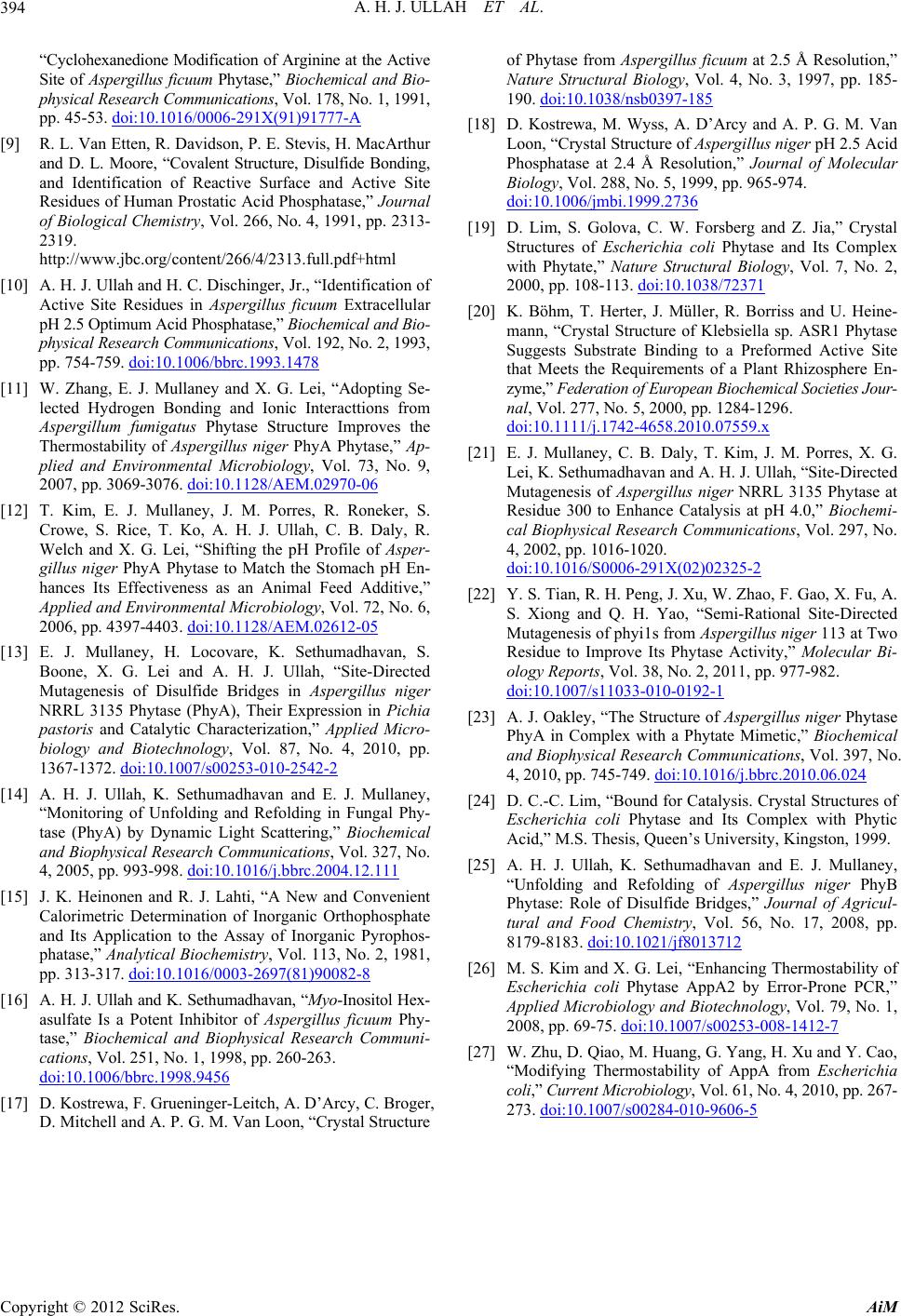
A. H. J. ULLAH ET AL.
394
“Cyclohexanedione Modification of Arginine at the Active
Site of Aspergillus ficuum Phytase,” Biochemical and Bio-
physical Research Communications, Vol. 178, No. 1, 1991,
pp. 45-53. doi:10.1016/0006-291X(91)91777-A
[9] R. L. Van Etten, R. Davidson, P. E. Stevis, H. MacArthur
and D. L. Moore, “Covalent Structure, Disulfide Bonding,
and Identification of Reactive Surface and Active Site
Residues of Human Prostatic Acid Phosphatase,” Journal
of Biological Chemistry, Vol. 266, No. 4, 1991, pp. 2313-
2319.
http://www.jbc.org/content/266/4/2313.full.pdf+html
[10] A. H. J. Ullah and H. C. Dischinger, Jr., “Identification of
Active Site Residues in Aspergillus ficuum Extracellular
pH 2.5 Optimum Acid Phosphatase,” Biochemical and Bio-
physical Research Communications, Vol. 192, No. 2, 1993,
pp. 754-759. doi:10.1006/bbrc.1993.1478
[11] W. Zhang, E. J. Mullaney and X. G. Lei, “Adopting Se-
lected Hydrogen Bonding and Ionic Interacttions from
Aspergillum fumigatus Phytase Structure Improves the
Thermostability of Aspergillus niger PhyA Phytase,” Ap-
plied and Environmental Microbiology, Vol. 73, No. 9,
2007, pp. 3069-3076. doi:10.1128/AEM.02970-06
[12] T. Kim, E. J. Mullaney, J. M. Porres, R. Roneker, S.
Crowe, S. Rice, T. Ko, A. H. J. Ullah, C. B. Daly, R.
Welch and X. G. Lei, “Shifting the pH Profile of Asper-
gillus niger PhyA Phytase to Match the Stomach pH En-
hances Its Effectiveness as an Animal Feed Additive,”
Applied and Environmental Microbiology, Vol. 72, No. 6,
2006, pp. 4397-4403. doi:10.1128/AEM.02612-05
[13] E. J. Mullaney, H. Locovare, K. Sethumadhavan, S.
Boone, X. G. Lei and A. H. J. Ullah, “Site-Directed
Mutagenesis of Disulfide Bridges in Aspergillus niger
NRRL 3135 Phytase (PhyA), Their Expression in Pichia
pastoris and Catalytic Characterization,” Applied Micro-
biology and Biotechnology, Vol. 87, No. 4, 2010, pp.
1367-1372. doi:10.1007/s00253-010-2542-2
[14] A. H. J. Ullah, K. Sethumadhavan and E. J. Mullaney,
“Monitoring of Unfolding and Refolding in Fungal Phy-
tase (PhyA) by Dynamic Light Scattering,” Biochemical
and Biophysical Research Communications, Vol. 327, No.
4, 2005, pp. 993-998. doi:10.1016/j.bbrc.2004.12.111
[15] J. K. Heinonen and R. J. Lahti, “A New and Convenient
Calorimetric Determination of Inorganic Orthophosphate
and Its Application to the Assay of Inorganic Pyrophos-
phatase,” Analytical Biochemistry, Vol. 113, No. 2, 1981,
pp. 313-317. doi:10.1016/0003-2697(81)90082-8
[16] A. H. J. Ullah and K. Sethumadhavan, “Myo-Inositol Hex-
asulfate Is a Potent Inhibitor of Aspergillus ficuum Phy-
tase,” Biochemical and Biophysical Research Communi-
cations, Vol. 251, No. 1, 1998, pp. 260-263.
doi:10.1006/bbrc.1998.9456
[17] D. Kostrewa, F. Grueninger-Leitch, A. D’Arcy, C. Broger,
D. Mitchell and A. P. G. M. Van Loon, “Crystal Structure
of Phytase from Aspergillus ficuum at 2.5 Å Resolution,”
Nature Structural Biology, Vol. 4, No. 3, 1997, pp. 185-
190. doi:10.1038/nsb0397-185
[18] D. Kostrewa, M. Wyss, A. D’Arcy and A. P. G. M. Van
Loon, “Crystal Structure of Aspergillus niger pH 2.5 Acid
Phosphatase at 2.4 Å Resolution,” Journal of Molecular
Biology, Vol. 288, No. 5, 1999, pp. 965-974.
doi:10.1006/jmbi.1999.2736
[19] D. Lim, S. Golova, C. W. Forsberg and Z. Jia,” Crystal
Structures of Escherichia coli Phytase and Its Complex
with Phytate,” Nature Structural Biology, Vol. 7, No. 2,
2000, pp. 108-113. doi:10.1038/72371
[20] K. Böhm, T. Herter, J. Müller, R. Borriss and U. Heine-
mann, “Crystal Structure of Klebsiella sp. ASR1 Phytase
Suggests Substrate Binding to a Preformed Active Site
that Meets the Requirements of a Plant Rhizosphere En-
zyme,” Federation of European Biochemical Societies Jour-
nal, Vol. 277, No. 5, 2000, pp. 1284-1296.
doi:10.1111/j.1742-4658.2010.07559.x
[21] E. J. Mullaney, C. B. Daly, T. Kim, J. M. Porres, X. G.
Lei, K. Sethumadhavan and A. H. J. Ullah, “Site-Directed
Mutagenesis of Aspergillus niger NRRL 3135 Phytase at
Residue 300 to Enhance Catalysis at pH 4.0,” Biochemi-
cal Biophysical Research Communications, Vol. 297, No.
4, 2002, pp. 1016-1020.
doi:10.1016/S0006-291X(02)02325-2
[22] Y. S. Tian, R. H. Peng, J. Xu, W. Zhao, F. Gao, X. Fu, A.
S. Xiong and Q. H. Yao, “Semi-Rational Site-Directed
Mutagenesis of phyi1s from Aspergillus niger 113 at Two
Residue to Improve Its Phytase Activity,” Molecular Bi-
ology Reports, Vol. 38, No. 2, 2011, pp. 977-982.
doi:10.1007/s11033-010-0192-1
[23] A. J. Oakley, “The Structure of Aspergillus niger Phytase
PhyA in Complex with a Phytate Mimetic,” Biochemical
and Biophysical Research Communications, Vol. 397, No.
4, 2010, pp. 745-749. doi:10.1016/j.bbrc.2010.06.024
[24] D. C.-C. Lim, “Bound for Catalysis. Crystal Structures of
Escherichia coli Phytase and Its Complex with Phytic
Acid,” M.S. Thesis, Queen’s University, Kingston, 1999.
[25] A. H. J. Ullah, K. Sethumadhavan and E. J. Mullaney,
“Unfolding and Refolding of Aspergillus niger PhyB
Phytase: Role of Disulfide Bridges,” Journal of Agricul-
tural and Food Chemistry, Vol. 56, No. 17, 2008, pp.
8179-8183. doi:10.1021/jf8013712
[26] M. S. Kim and X. G. Lei, “Enhancing Thermostability of
Escherichia coli Phytase AppA2 by Error-Prone PCR,”
Applied Microbiology and Biotechnology, Vol. 79, No. 1,
2008, pp. 69-75. doi:10.1007/s00253-008-1412-7
[27] W. Zhu, D. Qiao, M. Huang, G. Yang, H. Xu and Y. Cao,
“Modifying Thermostability of AppA from Escherichia
coli,” Current Microbiology, Vol. 61, No. 4, 2010, pp. 267-
273. doi:10.1007/s00284-010-9606-5
Copyright © 2012 SciRes. AiM