Modern Research in Catalysis
Vol.2 No.2(2013), Article ID:29896,10 pages DOI:10.4236/mrc.2013.22004
Liquid Phase Hydrogenation of p-Chloronitrobenzene on Au-Pd/TiO2 Catalysts: Effects of Reduction Methods
Department of Chemical and Materials Engineering, National Central University, Jhongli City, Chinese Taipei
Email: *ywchen@ncu.edu.tw
Copyright © 2013 Yu-Wen Chen, Der-Shing Lee. This is an open access article distributed under the Creative Commons Attribution License, which permits unrestricted use, distribution, and reproduction in any medium, provided the original work is properly cited.
Received December 3, 2012; revised January 10, 2013; accepted January 19, 2013
Keywords: Nanoalloy; Gold Catalyst; Au-Pd Catalysts; Hydrogenation of Chloronitrobenzene; Chloroaniline
ABSTRACT
The effects of palladium addition and the reduction methods on Au/TiO2 were investigated. Pd was loaded on TiO2 firstly by incipient-wetness impregnation, Au was then loaded by deposition-precipitation method. The nominal loadings of Au and Pd were 1 wt% and 0.01 wt%. The bimetallic catalysts were reduced by heating at 453 K, by flowing H2 at 423 K, or by NaBH4 at 298 K. The catalysts were characterized by ICP, XRD, TEM, HRTEM, TPR and XPS. Hydrogenation of p-chloronitrobenzene was carried out at 1.2 MPa H2 pressure and 353 K. The results showed that even adding very small amount of Pd could enhance activity and selectivity of p-chloroaniline significantly. Pd and Au formed alloy and Pd could donate partial electron to Au. Pd metal on the surface of alloy could adsorb hydrogen and enhanced the activity. The pretreatment methods did not change particle size significantly, all were below 4 nm. The sample reduced by NaBH4 could have higher concentration of Au0 and sustain small Au particle size, resulting in high activity.
1. Introduction
Halogenated aromatic amine is important intermediates for synthesis of organic fine chemicals, such as pesticides, herbicides, dyes, drugs and pigment, which were commercially target products. Conventional hydrogenation catalysts based on noble metal and nickel based catalyst [1-14], the noble metal such as palladium [15], platinum [16], rhodium [17] and ruthenium [18], which is limit their implementation to industrial applications because of cost effectiveness. In previous papers, one of the authors has reported that unsupported NiB catalysts are effective for hydrogenation of chloronitrobenzene [1-12].
Gold has been considered to be effective catalysts for hydrogenation reactions [19,20]. Due to its superior ability of hydrogenation adsorption, gold catalyst have many potential advantages of competitive selectivity and activity on hydrogenation reactions. The reactions included the hydrogenation of CO, CO2, NO, ethene, propene, crotoaldehyde, citral and etc. Palladium-gold bimetallic alloys have been found to provide both active and selective catalysts for a number of reactions including CO oxidation, cyclotrimerization of acetylene to benzene, vinyl acetate synthesis, selective oxidation of alcohols to aldehydes or ketones, oxidation of hydrogen to hydrogen peroxide, and hydrocarbon hydrogenation [21]. In addition, goldpalladium alloys provide rather ideal systems for fundamental study since gold and palladium are completely miscible in all proportions with only a slight lattice mismatch [22].
Cardenas-Lizana et al. have investigated various catalysts for gas phase hydrogenation of p-chloronitrobenzene (p-CNB) [23-28]. It has been demonstrated that the gas phase hydrogenation of p-CNB over Au supported on alumina [23] and titania [24] was 100% selective in terms of –NO2 group reduction to p-choroaniline (p-CAN). By investigating the influence of thin gold film surface nanostructure on chemisorption of hydrogen on unsintered gold films at 78 K, Stobinski et al. [29] have reported that H2 chemisorption occurs only on Au surface atoms of low coordination number forming AuH2 complexes. This suggests a dependence of the chemisorption process on the increasing amount of corners and edges for decreasing gold particle sizes. Since the adsorption capability of hydrogen on clean gold surface is limited, palladium was added in this study. Palladium has very strong ability to absorb up to 900 times its own volume of hydrogen at room temperatures. The addition of palladium adsorbed high amount of hydrogen provides active sites for hydrogen activation. Cardenas-Lizana et al. [25] reported that PdAu/Al2O3 is an active and selective catalyst for vapor phase hydrogenation of p-CNB. The only product was p-CAN. High Au and Pd loadings and alumina were used in their study. In addition, the vapor phase reaction was used which is different from liquid phase reaction.
In this study, the low Au and Pd metal loadings were used, TiO2 was used as the support instead of Al2O3, and the hydrogenation reaction was carried out in liquid phase. It has been reported that Au/TiO2 was more active than Au/Al2O3. Therefore TiO2 was chosen as the support in this study. In addition, it is known that the pretreatment method plays a role to determine the catalytic properties of Au, therefore the effects of pretreatment on the catalytic properties of PdAu/TiO2 were also investigated.
2. Experimental
2.1. Catalyst Preparation
Pd/TiO2 was prepared by incipient-wetness impregnation method with Pd(NO3)2 (Alfa Aesar, 99.9%) dissolved in distilled water. Pd(NO3)2 solution was added into TiO2 (Evonik-Degussa, P25) powder in drops under slowly stirring with 0.01 wt%. It was then calcined at 300˚C for 4 h. Gold catalysts were prepared by deposition-precipitation method (DP) with 1 wt% Au nominal loading. A suspension of the support in distilled water was placed in an ultrasound bath in order to disperse the support particles. The suspension was then placed in a flask, distilled water was added and the mixture was heated to 353 K at which a solution of HAuCl4 corresponding to the desired Au loading (1%, w/w), was added with a ca. 100-fold excess of NH4OH. The pH of the suspension was adjusted to 7. The solids obtained were separated by centrifugation, washed three times with deionized water. It was dried at 353 K for 16 h.
Au-Pd/TiO2 catalyst was reduced by three different ways. Catalyst was reduced either by heated at 180˚C, by H2 gas at 423 K, or by NaBH4 at ambient temperature. It is known that Au cations could be reduced by heating at 453 K. The temperature was high enough to reduce the cation, but not too high to cause sintering. Au-Pd/TiO2 represents for the sample heating at 453 K. In the second method, the catalyst was heated with flowing nitrogen at 373 K for 1 h to remove water, and then reduced with 10% H2/90% Ar gas mixture at 423 K for 2 h. In the third method, catalyst was reduced by sodium borohydride in the methanolic solution (50/50 methanol/water) at room temperature. The solution of sodium borohydride (1 M) was added into the solution very slowly under nitrogen stream to expel air in solution.
2.2. Characterization
The samples were dried under vacuum before carrying out the analysis of inductively-coupled plasma-mass spectrometry (ICP-MS), X-ray diffraction (XRD), transmission electron microscopy (TEM), high resolution transmission electron microscopy (HRTEM), energy dispersive spectrometer (EDS) and X-ray photoelectron spectroscopy (XPS).
The bulk compositions of the catalysts were analyzed by ICP-MS (PE/SCIEX Elan 6100 DRC). The ICP-MS was used for the reduction of plasma based interferences. To release mechanical stress from the inclusion, a small beam size was used to open the inclusion, followed by successive enlargement of the ablation pit. Hydrogen was used as reaction gas for the removal of plasma based interferences when operated at DRC mode. Quantitative results were calculated after calibration of the instrument response using SRM NIST 610 or 612 and a halite for Cl. Al-bulk concentrations, determined by electron microprobe, were used as internal standard in the case of the melt inclusions. The advantage from this gain in sensitivity is shown by the transient signal for a melt inclusion (40 μm diameter) from the Mole Granite. The comparison between standard and DRC operation mode shows an excellent agreement between the concentrations obtained for a series of fluid inclusions.
The XRD patterns were acquired on a Siemens D8-A powder diffractometer using Ni-filtered CuKα1 radiation (0.15418 nm) at a voltage and current of 40 kV and 40 mA, respectively. The sample was scanned over the range 2θ = 20˚ − 70˚ at a speed of 3˚/min to identify the crystalline structure. The samples for XRD were prepared as thin layers on a sample holder. Diffractograms were identified using the JCPDS-ICDD reference standards.
The morphologies and particle sizes of the samples were determined by transmission electron microscopy (TEM) on a JEM-2000 FX II (JEOL) operated at 120 kV and 160 kV. HRTEM was operated on a JEOL JEM- 2010 at 160 kV. Initially, a small amount of sample was fed into the sample bottle filled with a 99% methanol solution. After agitating under ultrasonic environment for 90 min, one drop of the dispersed slurry was dipped onto a carbon-coated copper mesh (300#) (Ted Pella Inc., CA, USA), and dried at room temperature in vacuum overnight. Au catalysts were measured in order to obtain a good statistical particle size distribution. TEM analysis was performed using a JEOL 100 CX II microscope. The particle diameters were measured from the enlarged photographs and the particle size distribution histograms were obtained on the measurements of at least 300 randomly selected individual nanoparticles. The size limit for the detection of gold particles on TiO2 was 1 nm.
XPS spectra were recorded with a Thermo VG Scientific Sigma Prob spectrometer. The XPS patterns were collected using AlKα radiation at a voltage and current of 20 kV and 30 mA, respectively. The sample was pressed into a self-supported disk before being mounted on a sample plate. Then it was degassed in the pretreatment chamber at 383 K for 2 h in vacuum before being transferred into the analyzing chamber. The base pressure in the analyzing chamber was maintained in the order of 2 × 10−9 torr. The spectrometer was operated at 23.5 eV pass energy. All the binding energy (BE) values were obtained after removing the surface oxides by Ar+ sputtering and were referenced to the contaminant carbon at C1s = 284.5 eV with an uncertainty of ± 0.2 eV. Peak fitting was done by using the software, XPSPEAK 4.1 with Shirley background, 30:70 Lorentzian to Gaussian convolution product shapes.
2.3. Catalytic Reaction
All the reaction experiments were carried out in a cylindrical stirred-tank reactor (Parr Instrument Model 4842). The reactor was charged by 0.002 mol Ni catalyst and 2.54 g p-CNB in 80 mL absolute methanol solvent, the concentration of p-CNB was 0.2 M. It was reported that methanol was a better reaction medium than ethanol for the hydrogenation reaction. The reactor was purged with hydrogen four times to expel air. During the run, the samples were withdrawn periodically (every 10 min) and analyzed by a gas chromatograph equipped with a flame ionization detector and a 3 m × 1/8 inch stainless steel column packed with 5% OV-101 on Chromsorb WAWDMSC (80 - 100 mesh). Repeated catalytic runs with different samples from the same batch of catalyst delivered product compositions that were reproducible to within ± 5%. The conversion and the selectivity to each product were calculated as following:
(1)
(2)
(3)
(4)
where CAN, CNB, Cp-CAN and Cp-CNB represented the concentration of aniline, nitrobenzene, p-CAN and p-CNB, respectively.
3. Results and Discussion
3.1. ICP-MS
The ICP-MS results shown in Table 1 reveal the amount of exact Au and Pd loadings on the TiO2 support. In this study, the nominal Au and Pd loadings were 1 wt% and 0.01 wt%, respectively. Only about 70% of Au and Pd were deposited on the support after DP process. The results are in agreements with literature data. Some of Pd was lost during DP process. The pretreatment did not influence the metal loadings significantly.
3.2. XRD
The XRD patterns of monometallic Au/TiO2 catalyst and bimetallic Au-Pd/TiO2 catalysts are show in Figure 1. All catalyst showed intense XRD peaks for antase phase at 2θ = 25.60˚(101), 38.03˚(004), 48.29˚(200), 53.94˚ (105), 55.05˚(211), 62.66(204) and 68.93˚(116) and rutile phase at 2θ = 27.45˚(110), 36.09˚(101) and 54.33˚ (54.33). TiO2 from Degussa has these two phases. The preparation and pretreatment did not change the structure of TiO2 as expected. No distinct XRD peaks for Au at 2θ = 38.2˚ and 44.5˚ were observed, because the particle size of gold was too small to detect. This confirms that Au particles were less than 4 nm. No peaks for Pd, PdO,
Figure 1. XRD patterns of the sample. (a) Au-Pd/TiO2; (b) Au-Pd/TiO2 reduced by H2; (c) Au-Pd/TiO2 reduced by NaBH4; and (d) Au/TiO2.
Table 1. Actual Au and Pd loadings of the catalysts.
and PdO2 were detected, which is attributed to the very low Pd loading (0.001 wt%).
3.3. TEM and HRTEM
Figure 2 shows the TEM micrographs and the corresponding gold particle size distribution of various AuPd/ TiO2 catalysts. The average Au particle size, dAu, was calculated by the following equation: where ni is the number of particles of diameter. The gold nanoparticles were homogeneously dispersed on TiO2 support. The particle size of TiO2 P-25 from Degussa is in the range of 20 - 40 nm. It constrained the sizes of Au and Pd particles deposited on it. Table 2 shows that the Au-Pd/TiO2 and Au/TiO2 heated at 453 K had the smallest average Au particle size. Adding Pd did not change the particle size of Au because it was small enough. The sample reduced by flowing hydrogen at 423 K had slightly larger Au particles. It is known that hydrogen atmosphere and high temperature could induce migration of metal atoms. Nevertheless, the difference was very small. One can conclude that the pretreatment almost did not change the Au particle size.
Figure 3 shows the diffraction rings of Au-Pd/TiO2
catalyst and Au-Pd/TiO2 reduced by NaBH4. The distance of lattice was calculated as follows:
(1)
where d represents the distance of lattice, radius represents the distance from the diffraction ring or pot to the center. These two diffraction images between Au-Pd/TiO2 heated at 453 K and Au-Pd/TiO2 reduced by NaBH4 reveal the same patterns. No palladium species was detected, which was attributed to the very low Pd loading (0.001 wt%), in consistent with the XRD results. Pd must be in very small particle size and did not form crystal itself in this study, Cardenas-Lizana et al. [25] have reported that Pd and Au formed nanoalloy. Our results are in accord.
The morphology, structure and orientation of the gold particles on Au-Pd/TiO2 and Au-Pd/TiO2 reduced by NaBH4 samples have been determined by HRTEM. The HRTEM images of all Au-Pd/TiO2 catalysts are shown in Figure 4. The uniform dispersion of gold particles with diameter less than 5 nm was observed. The gold particles were deposited on both rutile and anatase TiO2. Figure 4(a) shows the (111) plane of gold supported on the (112) plane of the anatase phase, which neared by the (111)
(a)
(b)
(c)
(d)
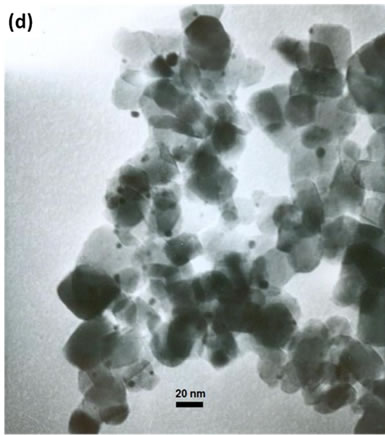
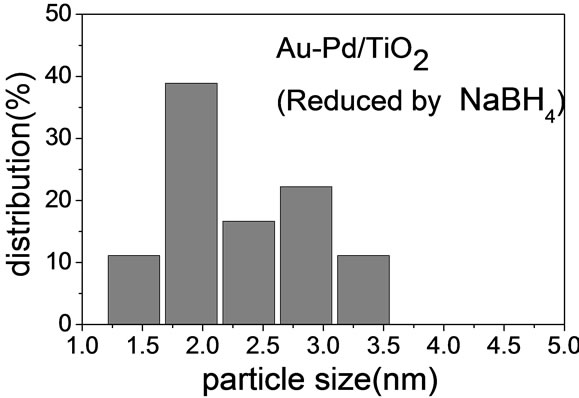
Figure 2. TEM images of the sample: (a) Au-Pd/TiO2; (b) Au-Pd/TiO2; (c) Au-Pd/TiO2 (reduced by H2); (d) Au-Pd/TiO2 reduced by NaBH4; (e) Au-Pd/TiO2 reduced by NaBH4 after reaction.
Table 2. The average of Au particle diameter (nm).
plane of rutile phase of the Au-Pd/TiO2 catalyst.
Figure 4(b) shows gold supported on the (200) plane of the rutile phase, which overlapped with the (101) plane of anatanse phase of the Au-Pd/TiO2 reduced by NaBH4. Figure 4(c) shows a 5 nm Au particle with (200) plane. It should be noted that no palladium species were detected, because the particle size was less than the detection limit (1 nm).
Figure 5 shows the mapping of Au-Pd/TiO2 at the specific position on the surface of this sample. Both Au and Pd were very uniformly distributed on TiO2 support.
3.4. XPS
The compositions and electronic structure of each species on the surface of the samples were determined by XPS analysis. The XPS spectra of Au 4f, Ti 2p and O 1s in the AuPd/TiO2 catalysts are shown in Figure 6.
The de-convolution of XPS spectra for all the catalysts suggests that Au species exists in different states as metallic gold (Au0) and Au3+ as shown in Figure 6. The Au 4f is characterized by the doublet of two spin orbit components, viz., Au 4f7/2 and Au 4f5/2. The peaks for metallic gold were centered at 84.0 eV (Au 4f7/2) and 87.7 eV (Au 4f5/2). The peaks for Au3+ located at 86.3 eV (Au 4f7/2) and 89.6 eV (Au 4f5/2). The Ti 2p XPS spectrum was deconvoluted into four peaks shown in Figure 6. The Ti 2p3/2 at 455 ± 0.3 eV is indicative of Ti3+, whereas the Ti 2p3/2 at 459 eV is indicative of Ti4+. The O 1s XPS spectrum was deconvoluted to two peaks as shown in Figure 6. The O 1s at 528 - 531 eV is indicative of metal oxides (O2−), whereas that at 531 - 532 eV is indicative of hydroxides (OH−). The deconvolution results are tabulated in Table 3. It should be noted that the Pd 3d signal was difficult to detect; Pd signal was very weak and overlapped with Au 4d5/2.
As shown in Table 3, the binding energy of Au changed with the addition of palladium and pretreatment condition. The binding energy of Au in AuPd/TiO2 was higher than that in Au/TiO2. The binding energy of Au shifted negatively in the AuPd/TiO2 samples reduced by NaBH4 and by H2. One could conclude that Pd donated part electron to Au, making Au more electron-enriched.
Ti 2p did not change by adding Pd and by different pretreatments, because the pretreatment temperature was
(a)
(b)
(c)
Figure 4. HRTEM image of (a) Au-Pd/TiO2; (b) Au-Pd/ TiO2 reduced by NaBH4; (c) Au-Pd/TiO2 reduced by H2.
not high enough to change its state. No Ti3+ was detected, indicating that TiO2 structure was very stable under preparation and reduction conditions. Table 4 shows the per-
Figure 5. EDS spectra of Au-Pd/TiO2.
Table 3. Binding energies of the species on the surface of the catalyst.
Table 4. The compositions of the species on the surface of the catalyst.
Table 5. Effect of additives on the hydrogenation of p-CNB over Au-Pd/TiO2 series catalystsa.
(a)
(b)
(c)
Figure 6. XPS spectra.
centages of various species on the surface of the catalysts. AuPd/TiO2 reduced by NaBH4 had the highest concentration of metallic Au species. It also had the highest concentration of hydroxyl groups on the surface.
3.5. Hydrogen Reaction
In order to obtain the intrinsic kinetic data, it is important to ensure that the rate data are obtained under the kinetically controlled regime. In the liquid phase hydrogenation reactions, gas-liquid, liquid-solid and intraparticle diffusion resistances are likely to be important for consumption of hydrogen. A direct test of the important of gasliquid and liquid-solid transport was made by running the several experiments at different agitation speeds, with all other variables constant. The initial rates were found to increase significantly when the agitation speed increased from 0 to 250 rpm, but it remained constant after 300 pm. It appears from these results that the resistance to gasliquid and liquid-solid mass transfer is not important above 300 rpm. All of the experiments were thus conducted at an agitation speed of 500 rpm. The intraparticle mass transfer in any porous solid-catalyzed reaction can be eliminated by using the small particle size <45 μm. In the present study the catalyst particle size was <30 μm and hence intraparticle mass transfer resistance was assumed to be insignificant. To confirm the absence of intraparticle mass transfer diffusion, we calculated the value of effectiveness factor. The values are close to 1 in all the experimental runs, therefore, we concluded that the intraparticle mass transfer diffusion was not significant.
Pretreatment is important for the activity of Au-Pd/ TiO2 catalyst. The pretreatment conditions should be rigorous enough to yield bare Au-Pd alloy, but not so harsh that the resulting Au-Pd particles are too large. Figure 7" target="_self"> Figure 7 shows the conversion of p-CNB and selectivity of pCAN vs. reaction time. It illustrates that the activity decreased in the following order: Au-Pd/TiO2 reduced by NaBH4 > Au-Pd/TiO2 heated at 453 K ≧ Au-Pd/TiO2 reduced by H2 ≧ Au/TiO2. The reaction was first-order with respect to the concentration of p-CNB. All the bimetallic catalysts had higher activities and selectivities than the monometallic Au/TiO2 catalyst even only very small amount of Pd was added. The results clearly inferred that Au and Pd form alloy and had synergistic effect. Au-Pd/TiO2 reduced by NaBH4 had the highest conversion of p-CNB. Since p-CAN is the intermediate product in a series of reactions, it is expected that the selectivity of p-CAN is lower if the catalyst is too active. The sample heated at 453 K had smaller Au particles than that of Au-Pd/TiO2 reduced by hydrogen. Au-Pd could be reduced by heating at 453 K. The presence of hydrogen could increase the mobility of metals, resulting in aggregation of metals. The Au-Pd/TiO2 reduced by H2 had the lower activity than that reduced by NaBH4. The reduction temperature in flowing hydrogen was set at 423 K to avoid sintering of gold particles, but temperature might be too low to completely reduce the metal. Au-Pd/TiO2 reduced by H2 presented the lower conversion and selective than Au-Pd/TiO2 reduced by NaBH4 and Au-Pd/ TiO2 heat at 453 K. Proper reduction treatment of Au-Pd/ TiO2 could enhance the activity and selectivity greatly.
Since the adsorption capability of hydrogen on gold
(a)
(b)
Figure 7. (a) p-CNB conversion and (b) p-CAN selectivity (Reaction condition: 0.05 g catalysts, 2.54 g of p-CNB, 80 ml of methanol, 353 K, H2 pressure of 1.2 MPa, stirring speed of 500 rpm).
surface is limited [29], Pd was added in this study. Palladium has the uncommon ability to absorb up to 900 times its own volume of hydrogen at room temperatures [25]. Palladium provided active sites for hydrogen activation of Au-Pd/TiO2 and adsorbed high amount of hydrogen. Pd donated part electron to Au which also could enhance the adsorption of hydrogen on Au. Both are beneficial for hydrogenation [25]. In the hydrogenation of p-CNB, since part of electron transferred from Pd to Au facilitated the catalyst attracting the partial negative oxygen easily so the N=O bond of –NO2 group was activated, and the hydrogen could attack the partial positive nitrogen easily [25]. This behavior accelerated the completion of hydrogenation reaction. Combination of the electron effects and the structure effects, the Au-Pd/TiO2 catalyst was more active after reduction by sodium borohydride rather than by H2. The promotional effect of Pd has resulted in a significant increase in hydrogenation rate while still retaining the selectivity due to Au.
Scheme 1. Reaction mechanism of p-CNB hydrogenation.
In this study, the main product of p-CNB hydrogenation was p-CAN and there were two by-products, nitrobenzene (NB) and aniline (AN). The simplified reaction route is displayed in Scheme 1. In each case, the concentration of NB and AN increased at first, then decreased and AN would become zero eventually. There are two possible explanations: one is that p-CNB would mainly follow path (1) and path (2) but less to path (3) and path (4); another is that the rate of the hydrogenation carried on the path (1) is faster than the path (3). However, the increasing rate of aniline was not obvious, so the possibility of the latter case is low.
The binding energy of Au shifted to a lower value in Au-Pd/TiO2 reduced by NaBH4 catalyst, indicating that Au received more electrons from other elements. Based on the electronic effect, both –NO2 and –Cl adsorb strongly on electron-rich metal, which would result in higher activity in the hydrogenation of the nitro group and de-chlorination [8-10]. Higher electron density on Au could facilitate the formation of H− species and activating the adsorbed –NO2 group through a back electron-donation from d-orbital of Au [15,16], which could promote p-CNB hydrogenation. It would result in strong adsorption of p-CNB on the active site of the elemental Au on the catalyst surface, and increased the activity of hydrogenation.
The nitro group consists of two highly electronegative elements, N and O. Oxygen atom is even more electronegative than nitrogen atom; hence, the N-O bond is polarized. The partially positive charge of nitrogen atom, combined with high electronegativity, makes the nitrogroup easily reducible. The para-substituted nitro group has the high electronegativity resulting from the combination of both inductive and resonance effects. For chorine, only the inductive effect is present. Since the –NO2 group is more electronegative than –Cl, -NO2 is supposed to occupy the active site on the gold particles at the start of the reaction. The –NO2 group adsorbed on the catalyst surface is hydrogenated to from p-CAN which is further desorbed. When –Cl pre-occupied the catalyst surface, hydrogenation followed by dehalogenation occurred, leading to the formation of nitrobenzene which is eventually desorbed [30]. It is possible that the desorbed nitrobenzene would re-adsorb on the catalyst surface. As a result of the chemical bonding in the nitro group, the nitrogen atom is positively charged and each oxygen atom has a partial negative charge. For this reason the nitro group strongly attracts electrons. Since the binding energy of Au 4f7/2 was negatively shifted in Au-Pd/TiO2 reduced by H2, the gold on the surface became more electron-enriched. The high electronegativity of –NO2 would be adsorbed on electro-rich gold, which would result in higher activity in the hydrogenation of the nitro group and dechlorination. These results establish the viability of Pd promoted selective –NO2 reduction over supported Au.
The results presented here have shown that Au-Pd/ TiO2 catalyst is a promising catalyst for industrial application. The best reduction method is by sodium borohydride.
3.6. Reaction Rate Constant
One could calculate the reaction rate constant of each reaction for the monometallic Au/TiO2 catalyst and bimetallic Au-Pd/TiO2 catalysts. Based on the conversiontime curves in the hydrogenation of p-CNB (Figure 7), it shows that the reaction was first order with respect to the concentration of p-CNB. The reaction rate can be expressed as following:
(1)
where k is the reaction rate constant (s−1), CA0 is the initial concentration of reactant, and XA is the conversion of reactant (%).
The reaction was carried out in a constant-volume batch reactor, so:
(2)
where t is the reaction time (s). Combining the above two equations by using integral method of analysis of data, one could get:
(3)
A plot of vs. t could derive the slope which represents the reaction rate constant. Because of the induction period appeared in the initial stage of reaction, the data in initial stage was neglected. The results are shown in Table 5. The rate constant of Au-Pd/TiO2 reduced by NaBH4 was the highest among all the samples in this study.
4. Conclusion
Bimetallic Au-Pd/TiO2 is superior to monometallic Au/ TiO2 in selective hydrogenation of p-chloronitrobenzene for p-chloroaniline, which can be accounted for by the dominant population of the strongly bound hydrogen on Pd and the unique electronic structure of Au metal. The results showed that even adding very small amount of Pd could enhance activity and selectivity of p-chloroaniline significantly. Pd and Au formed alloy and Pd could donate part electron to Au. Pd metal on the surface of alloy could adsorb hydrogen and enhanced the activity. The pretreatment methods did not change particle size significantly, all were below 4 nm. The sample reduced by NaBH4 could have higher concentration of Au0 and sustain small Au particle size, resulting in high activity.
5. Acknowledgements
This research was supported by the Ministry of Economic Affairs, Taiwan.
REFERENCES
- Y. C. Liu and Y. W. Chen, “Hydrogenation of p-Chloronitrobenzene on La-promoted NiB Nanometal Catalysts,” Industrial and Engineering Chemistry Research, Vol. 45, No. 9, 2006, pp. 2973-2980. doi:10.1021/ie0509847
- Y. C. Liu, C. Y. Huang and Y. W. Chen, “Hydrogenation of p-choloronitrobenzene Ni-B on Nanometal Catalysts,” Journal of Nanoparticle Research, Vol. 8, No. 2, 2006, pp. 223-234. doi:10.1007/s11051-005-5944-9
- L. F. Chen and Y. W. Chen, “Effect of Additive (W, Mo, and Ru) on Ni-B Amorphous Alloy Catalyst in Hydrogenation of p-Chloronitrobenzene,” Industrial and Engineering Chemistry Research, Vol. 45, No. 26, 2006, pp. 8866-8873. doi:10.1021/ie060751v
- W. J. Wang, J. H. Shen and Y. W. Chen, “Hydrogenation of p-Chloronitrobenzene on Ni-P-B Nanoalloy Catalysts,” Industrial and Engineering Chemistry Research, Vol. 45, No. 26, 2006, pp. 8860-8865. doi:10.1021/ie0605736
- J. H. Shen and Y. W. Chen, “Catalytic Properties of Bimetallic NiCoB Nanoalloy Catalysts for Hydrogenation of p-Chloronitrobenzene,” Journal of Molecular Catalysis A: Chemical, Vol. 273, No. 1-2, 2007, pp. 265-276. doi:10.1016/j.molcata.2007.04.015
- Y. W. Chen and N. Sasirekha, “Preparation of NiFeB Nanoalloy Catalysts and Its Applications in Liquid Phase Hydrogenation of p-Chloronitrobenzene,” Industrial and Engineering Chemistry Research, Vol. 48, No. 13, 2009, pp. 6248-6255. doi:10.1021/ie801624a
- Y. W. Chen, N. Sasirekha and Y. C. Liu, “Hydrogenation of p-Chloronitrobenzene over NiPtB Nanoalloy Catalysts,” Journal of Non-Crystalline Solids, Vol. 355, No. 22-23, 2009, pp. 1193-1201.
- M. H. Lin, B. Zhao and Y. W. Chen, “Hydrogenation of p-Chloronitrobenzene over Mo-modified NiCoB Nanoalloy Catalysts: Effect of Mo Content,” Industrial and Engineering Chemistry Research, Vol. 48, No. 15, 2009, pp. 7037-7043. doi:10.1021/ie900019d
- B. Zhao and Y. W. Chen, “Hydrogenation of p-Chloronitrobenzene on Mo, La, Fe, and W-Modified NiCoB Nanoalloy Catalysts,” Journal of Non-Crystalline Solids, Vol. 356, No. 18-19, 2010, pp. 839-847.
- B. Zhao, C. J. Chou and Y. W. Chen, “Hydrogenation of p-Chloronitrobenzene on Tungsten-Modified NiCoB Catalyst,” Industrial and Engineering Chemistry Research, Vol. 49, No. 4, 2010, pp. 1669-1676. doi:10.1021/ie901606b
- B. Zhao and Y. W. Chen, “The Effect of Poly-N-vinylpyrrolidone Modification on NiCoB Catalysts for Hydrogenation of p-Chloronitrobenzene,” Materials Chemistry and Physics, Vol. 125, No. 3, 2011, pp. 763-768. doi:10.1016/j.matchemphys.2010.09.061
- J. F. Su, B. Zhao and Y. W. Chen, “Hydrogenation of pChloronitrobenzene on Mo-Doped NiB Cluster Catalysts,” Industrial and Engineering Chemistry Research, Vol. 50, No. 3, 2011, pp. 1580-1587. doi:10.1021/ie1016865
- Y. W. Chen and D. S. Lee, “Hydrogenation of p-Chloronitrobenzene on Nanosized Modified NiMoB Catalysts,” Catalysis Surveys from Asia, Vol. 16, No. 4, 2012, pp. 198-209. doi:10.1007/s10563-012-9144-1
- C. P. Li, Y. W. Chen and W. J. Wang, “Nitrobenzene Hydrogenation over Aluminum Borate-supported Platinum Catalyst,” Applied Catalysis A: General, Vol. 119, No. 2, 1994, pp. 185-194. doi:10.1016/0926-860X(94)85190-5
- R. Baltzly and A. R. Phillips, “The Catalytic Hydrogenolysis of Halogen Compounds,” Journal of American Chemical Society, Vol. 68, No. 2, 1946, pp. 261-265. doi:10.1021/ja01206a034
- J. R. Kosak, “Catalytic Hydrogenation of Aromatic Halonitro Compounds,” Annals of the New York Academy of Sciences, Vol. 172, 1970, pp. 175-186. doi:10.1111/j.1749-6632.1970.tb34975.x
- M. M. Dell’Anna, V. Gallo, P. Mastrorilli and G. Romanazzi, “A Recyclable Nanoparticle-Supported Rhodium Catalyst for Hydrogenation Reactions,” Molecules, Vol. 15, No. 5, 2010, pp. 3311-3318. doi:10.3390/molecules15053311
- M. Liu, W. Yu, H. Liu and J. Zheng, “Preparation and Characterization of Polymer-Stabilized Ruthenium-Platinum and Ruthenium-Palladium Bimetallic Colloids and Their Catalytic Properties for Hydrogenation of o-Chloronitrobenzene,” Journal of Colloid and Interface Science, Vol. 214, No. 2, 1999, pp. 231-240. doi:10.1006/jcis.1999.6186
- P. A. Sermon, G. C. Bond and P. B. Wells, “Hydrogenation of Alkenes over Supported Gold,” Journal of the Chemical Society, Faraday Transactions 1: Physical Chemistry in Condensed Phases, Vol. 75, 1979, pp. 385-392. doi:10.1039/f19797500385
- R. S. Yolles, B. J. Wood and H. Wise, “Hydrogenation of Alkenes on Gold,” Journal of Catalysis, Vol. 21, No. 1, 1971, pp. 66-72. doi:10.1016/0021-9517(71)90121-7
- M. Haruta, “Gold as a Novel Catalyst in the 21st Century: Preparation, Working Mechanism and Application,” Gold Bulletin, Vol. 37, No. 1-2, 2004, pp. 27-54. doi:10.1007/BF03215514
- Z. Li, F. Gao, Y. Wang, F. Calaza, L. Burkholder and W. T. Tysoe, “Formation and Characterization of Au/Pd Surface Alloys on Pd(111),” Surface Science, Vol. 601, No. 8, 2007, pp. 1898-1908. doi:10.1016/j.susc.2007.02.028
- F. Cárdenas-Lizana, S. Gómez-Quero and M. A. Keane, “Ultra-selective Gas Phase Catalytic Hydrogenation of Aromatic Nitro Compounds over Au/Al2O3,” Catalysis Communications, Vol. 9, No. 3, 2008, pp. 475-482. doi:10.1016/j.catcom.2007.07.032
- F. Cárdenas-Lizana, S. Gómez-Quero and M. A. Keane, “Exclusive Production of Chloroaniline from Chloronitrobenzene over Au/TiO2 and Au/Al2O3,” Sustainable Chemistry & Green Chemistry, Vol. 1, No. 3, 2008, pp. 215- 223. doi:10.1002/cssc.200700105
- F. Cardenas-Lizana, S. Gomez-Quero, A. Hugon, L. Delannoy, C. Louis and M. A. Keane, “Pd-Promoted Selective Gas Phase Hydrogenation of p-Chloronitrobenzene over Alumina Supported Au,” Journal of Catalysis, Vol. 262, No. 2, 2009, pp. 235-243. doi:10.1016/j.jcat.2008.12.019
- F. Cardenas-Lizana, S. Gomez-Quero and M. A. Keane, “Gas Phase Hydrogenation of m-Dinitrobenzene over Alumina Supported Au and Au-Ni Alloy,” Catalysis Letters, Vol. 127, No. 1-2, 2009, pp. 25-32. doi:10.1007/s10562-008-9660-9
- F. Cardenas-Lizana, S. Gomez-Quero, N. Perret and M. A. Keane, “Support Effects in the Selective Gas Phase Hydrogenation of p-Chloronitrobenzene over Gold,” Gold Bulletin, Vol. 42, No. 2, 2009, pp. 124-132. doi:10.1007/BF03214922
- F. Cardenas-Lizana, S. Gomez-Quero and M. A. Keane, “Clean Production of Chloroanilines by Selective Gas Phase Hydrogenation over Supported Ni Catalyst,” Applied Catalysis A: General, Vol. 334, No. 1-2, 2008, pp. 199- 206. doi:10.1016/j.apcata.2007.10.007
- C. Y. Wang, C. Y. Liu, X. Zheng, J. Chen and T. Shen, “The Surface Chemistry of Hybrid Nanometer-Sized Particles: I. Photochemical Deposition of Gold on Ultrafine TiO2 Particles,” Colloids and Surfaces A: Physicochemical and Engineering Aspects, Vol. 131, No. 1-3, 1998, pp. 271-280. doi:10.1016/S0927-7757(97)00086-1
- Z. K. Yu, S. J. Liao, Y. Xu, B. Yang and D. R. Yu, “Hydrogenation of Nitroaromatics by Polymer-Anchored Bimetallic Palladium-Ruthenium and Palladium-Platinum Catalysts under Mild Conditions,” Journal of Molecular Catalysis A: Chemical, Vol. 120, No. 1-3, 1997, pp. 247-253. doi:10.1016/S1381-1169(96)00420-7
NOTES
*Corresponding author.