New Journal of Glass and Ceramics
Vol.4 No.1(2014), Article ID:42570,10 pages DOI:10.4236/njgc.2014.41002
CaCo1−xRuxOy: Role of Ru/Co Ratio on Its Transport Properties
1Vitreous State Laboratory, the Catholic University of America, Washington DC, USA; 2Currently at Corninng Incorporated, Corning, NY, USA; 3Currently at Naval Research Lab, Washington DC, USA.
Email: *annamalas@corning.com
Copyright © 2014 Sezhian Annamalai et al. This is an open access article distributed under the Creative Commons Attribution License, which permits unrestricted use, distribution, and reproduction in any medium, provided the original work is properly cited. In accordance of the Creative Commons Attribution License all Copyrights © 2014 are reserved for SCIRP and the owner of the intellectual property Sezhian Annamalai et al. All Copyright © 2014 are guarded by law and by SCIRP as a guardian.
Received May 12th, 2013; revised June 12th, 2013; accepted June 19th, 2013
Keywords:Calcium Cobaltite; Ruthenium Oxide; Calcium Ruthenate; Transport Properties; Thermoelectric Properties; Seebeck Coefficient; Resistivity
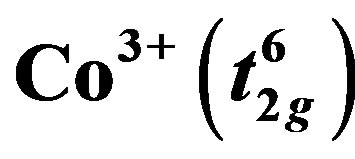
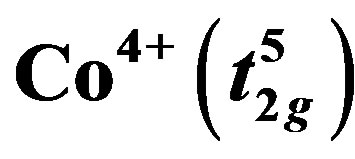
1. Introduction
Ceramic materials comprising transition metal oxides exhibit a number of unusual and technologically important phenomena, such as high temperature superconductivity in cuprates, colossal magnetoresistance in manganites, and superior thermoelectric properties in layered cobaltites [1,2]. Among these materials, the layered cobaltite with the composition Ca3Co4O9 exhibits metallic conductivity and extraordinarily large positive thermopower (s = 125 µV/K), which demonstrates that dominant carriers in Ca3Co4O9 are holes [3]. Ca3Co4O9 has a two-dimensional (2d) layered structure, which consists of alternating stacks of CaO-CoO-CaO rock salt type and CdI2-type hexagonal CoO2 layers along the c axis [1,4-7]. Both subsystems have identical a and c but different b parameters. The misfit between the two subsystems leads to an incommensurate spatial modulation along the b axis. The Ca3Co4O9 system exhibits very complicated magnetic and transport properties due to this complex crystal structure [8]. Although the compound has two crystallographically distinct Co ion sites, electric conduction mainly occurs within the CoO2 sheets because the other Co ion site is situated in the highly oxygen-deficient Ca2CoO3.4 subsystem [5].
Another example of cobalt oxides, the one dimensional (1-d) cobaltite, Ca3Co2O6, is also very attractive for its magnetic properties [1]. The rhombohedral structure of this phase [1] consists of [Co2O6] chains running along the c axis of the hexagonal cell. In each chain, one CoO6 octahedron alternates with one CoO6 trigonal prism. In these compounds the oxygen stoichiometry controls the redox or mixed valence state of the Co ions. Maignan et al., [9] demonstrated that in polycrystalline compounds (sintered in air under similar conditions), the oxygen stoichiometry increases with the size of the lanthanide ion.
Compounds containing RuO2 (commonly called ruthenates) have been observed to exhibit strong electronelectron correlation and a number of physical properties such as metal-insulator transition and antiferromagnetism in Ca2RuO4 [10,11], ferromagnetism in SrRuO3, and Sr4Ru3O10 [12,13], superconductivity in Sr2RuO4 [14] and metamagnetism and magnetoresistivity in Ca3Ru2O7 [15]. Among the layered ruthenates, the physical properties of Ca2RuO4 and CaRuO3 have been investigated earlier [16-18]. Another family of compounds with the general composition CaMO3 (M=Ru and Fe) has evoked considerable interest on account of their unusual magnetic properties which indicate paramagnetic behavior down to 30 mK [19-22]. This compound, CaRuO3, is composed of an array of corner-shared RuO6 octahedra and it is assumed that the degree of tilting and rotation of these octahedra within their ideal cubic-perovskite structure governs the magnetic and also the transport properties. In CaRuO3, a narrow itinerant 4d band is formed through hybridization of Ru-t2g and O-2p orbitals. The 4d bandwidth thus formed sensitively depends on degree of hybridization [23] which also determines the mobility of carriers, leading to electric conduction.
To optimize the carrier concentration and to improve the electrical transport of calcium cobaltite, one approach is to partially substitute Ca with alkali metals, alkaline earth metals, or rare-earth metals [24]. Another route towards improvement of the transport properties is the partial substitution of other 3d transition metals for Co [25]. The spin state of a transition metal ion, such as Co, is determined by the competition between the crystal field and intra-atomic Coulomb repulsion energy. Among the 3d transition metal elements, Co has both intra-atomic Coulomb energy and crystal field energy. The variation in spin and oxidation states of cobalt oxide allows them to be influenced by other 3d or 4d transition ions. The dshells of 4d transition metal ions are more extended than their 3d counterparts. This extended nature of 4d electrons enhances orbital overlapping which is expected to influence the transport and thermopower characteristics by affecting the spin state of the 3d Co ions. As discussed earlier, the spin state of Co ions has a large influence on the thermopower of these cobaltites.
In the present investigation, transport properties such as resistivity and thermopower of CaCo1−xRuxOy(CCR) have been determined as Co is substituted by Ru ions (up to 33 mol%), keeping CaO invariant at 50 mol%. Two samples (CCR-3 and CCR-4) were also prepared by replacing Co by Ca and Ca by Ru, respectively. X-ray diffraction (XRD) analysis, supported by electron microscopic investigation, has been presented to aid in the understanding of the variation in transport properties as a function of composition. The evolution of the structure as a function of TIR has been studied as the structure changes from needle shaped crystals for Co rich compositions to planar structure for Ru rich compositions. The thermopower and resistivity data have been discussed in the light of the general properties exhibited by calciumcobaltites and CaRuO3.
2. Experimental Procedure
Samples with the general composition CaCo1−xRuxOy, with x varying from 0.06 to 0.33, were prepared, as listed in Table 1. For compositions CCR-1, -2, -5 and -6, CaO was kept invariant at 0.5 moles and a gradual equimolar substitution of Co by Ru was made with Ru varying between 0.06 and 0.33. The composition represented by CCR-4 is a variation of CCR-6 in which an equimolar replacement of CaO by RuO2 was made while the compound designated CCR-3 is a variation of CCR-2 with an equimolar substitution of CoO by CaO.
All the CaCo1−xRuxOy samples were prepared, using reagent grade CaCO3, CoO and RuO2, following a threestage mixing protocol to ensure compositional homogeneity. In the first step, stoichiometric amounts of CaCO3, CoO and RuO2 were weighed using a Metler balance to an accuracy of 1 mg and mixed thoroughly by grinding them in an alumina mortar and pestle. The mixture was subsequently calcined in a muffle furnace, in ambient atmosphere, at 873 K for 4 hours, at a heating rate of 200 K/hr. in an alumina crucible. The calcined mixture was ground/mixed in a mortar and pestle and it was re-calcined in the same alumina crucible at a higher temperature (1073 K) using the same heating and cooling protocol. The purpose of repeated calcinations is to completely remove moisture and all the combustion products, especially the CO2 gas generated by the decomposition of CaCO3. After the second calcination step the samples were homogenized one last time, again by grinding in a mortar and pestle. This homogenized sample was then formed into pellets, 1 cm in dia and ~5 mm in thickness, by dry-pressing in a hydraulic press under an applied load of 35 MPa. These pellets were sintered at 1173 K for 10 hours on a fresh alumina substrate using the same heating protocol and were cooled to room temperature at a rate of 100 K/hr. The purpose of this elaborate procedure for sample preparation was to achieve reproducibility and compositional homogeneity of the samples which was confirmed by XRD and x-ray fluorescence spectroscopy (XRF) measurements.
Table 1. Composition and transport properties of CaCo1−xRuxOy Samples.
Several identical pellets of each CaCo1−xRuxOy composition were prepared as described above. One such pellet would be crushed into powder for XRF analysis for confirmation of its composition and the same powder would then be used as the sample for XRD analysis (Thermo ARL-X’tra Diffractometer), using Cu-Kα radiation. XRD data were analyzed by a commercial software (Jade) in the pattern-matching mode to identify the phase composition of the samples. Another pellet would be mounted using epoxy resin and then surface polished for microstructural and compositional analyses using scanning electron microscopy (SEM) with Energy Dispersive Spectroscopy (EDS) analysis (JEOL, JXA-35A) to verify the homogeneity of the prepared samples and detect if multiple phases were present in the samples.
Electrical conductivity was measured by the van der Pauw’s four-probe method [26].
The sintered CaCo1−xRuxOy samples were ground and polished to be coplanar with a thickness of about 1 - 2 mm. A fully computerized apparatus, capable of scanning between 82 and 700 K (H-50 and K-20 systems, MMR Technologies, Mountain View, California) was employed for this purpose and these resistivity measurements were conducted in a dark chamber, maintained at 8 - 10 mtorr vacuum, under ohmic conditions. The procedure used for electrical resistivity measurement on similar samples has been described in detail elsewhere [27]. In all, twenty four resistivity measurements were performed at each temperature and the average of these values comprises each of the resistivity data presented in Table 1. The error in temperature measurement was determined at ±0.5 K and the error margin of conductivity measurements is estimated at ±7%.
Thermopower (Seebeck coefficient) of the CaCo1−xRuxOy samples was measured with a computerized apparatus (SB-100 and K-20, MMR Technologies, Mountain View, California) and Figure 1 shows a sche-
Figure 1. Schematic of seebeck coefficient measurement system. a—heat sink, b & c—heat source, d—heater, e—reference constantan wire, f—sample.
matic description of this experimental setup. The procedure used for thermopower measurement on similar samples has been described in detail elsewhere [27]. The error in temperature determination was estimated at ±0.1 K and that of thermopower measurement is determined at ±10%. It is important to ensure good thermal contact (not just electrical contact) between the sample/reference wire and the heat sink/source copperpads.
3. Results and Discussion
Compositions of the six CaCo1−xRuxOy(CCR) samples prepared have been verified by XRF and are listed in Table 1. As discussed earlier the compositions represented by CCR-3 and -4 are variations of CCR-2 and -6 respectively in which an equimolar replacement of CaO by RuO2 or CoO by CaO was made. The measured properties of these samples (CCR-3, -4) are shown in italics in Table 1 and as diamonds in the figures to distinguish them from the rest of the compositions.
When TIR = 0, the sample composition is basically that of binary calcium cobaltite (CC-1, [28]). The sample composition turns out to be the binary calcium ruthenate (CaR-5), when TIR = 1. All the regular CaCo1–xRuxOy samples (CCR-1, -2, -5 and -6) fall between these two end members. In the following the properties of binary calcium ruthenate (CaR-5, [27]) and binary calcium cobaltite (CC-1) will be compared with the ternary CaCo1−xRuxOy compositions.
The resistivity data of the CaCo1−xRuxOy samples are presented in Figure 2 as a function of temperature (ln ρ vs 1000/T), which shows that the resistivity decreases with increasing temperature, 300 to 600 K. The resistivity values of the CaCo1−xRuxOy samples measured at 600 K provided in Table 1 and Figure 3 depict the effect of Ru substitution, in the form of TIR, on the resistivity of the CaCo1−xRuxOy system. The resistivity of CaR-5, the binary calcium ruthenate [27] is less than that of CC-1 which is the binary calcium cobaltite [28]. A monotonic reduction in resistivity is expected, in non-metallic systems, and observed as RuO2 is added to the samples and the composition moves from CC-1 to CaR-5. This monotonic reduction in resistivity with Ru addition is in line with results found in literature for similar systems [29]. The explanation offered being that the charge carriers in these systems are the electrons occupying the d-orbitals of Ru and Co ions and even though Ru and Co are both transition ions, the outer orbital of the former comprises 4d electrons whereas that of Co is occupied by 3d electrons. 4d orbitals have larger overlapping characteristics than their 3d counterparts and result in greater mobility of the charge carriers. As a result the resistivity decreases with increasing proportion of RuO2 in the composition. The resistivity decreases from 8.24 × 10−4 Ω∙m for CCR- 6 (TIR = 0.12) to 1.42 × 10−4 Ω∙m for CCR-1 (TIR = 0.66).
Comparing the resistivities of CCR-4 and -6, it was observed that replacement of Ca by Ru ions reduces resistivity, which can be explained by the extended nature of the Ru 4d electron orbitals and this reduction in resistivity is in line with the results found in literature for similar systems, for example calcium manganites [1,29]. Conversely, comparing the resistivities of CCR-2 and -3, it was observed that replacement of Co by Ca increases the resistivity and this increase in resistivity is expected since alkaline earth ions have rather constrained outer orbitals compared to those of 3d transition ions such as Co.
Samples which have a TIR ≥ 0.5, CCR-1 and -2, have resistivity values similar to that of CaR-5, pure calcium ruthenate as can be seen from Table 1 and Figure 3. Similar behavior is observed in calcium ruthenates when either Ca is replaced by other alkaline earths or the Ru ions by lanthanides [29]. The other two samples CCR-5 and -6 have higher resistivity and closer to that of CC-1 composition.
The thermopower data of the CaCo1−xRuxOy samples (CCR-1, 2, 5 and 6) are presented in Figure 4 as a function of temperature, which shows that for all the compo-
Figure 2. Electrical resistivity of ternary CaCo1−xRuxOy samples as a function of temperature with resistivity decreasing with increasing temperature for all the samples.
Figure 3. Electrical resistivity, at 600 K, of ternary CaCo1−xRuxOy samples as a function of TIR showing a decrease in resistivity with increasing TIR.
sitions S increases with increasing temperature, in the range of 300 to 600 K. The CaCo1−xRuxOy samples with TIR ≥ 0.5 do not show any significant change in S with temperature over the 300 K range, while the ternary samples with TIR < 0.5 do exhibit an increase in S with temperature over the same temperature range. The Seebeck coefficient at 600 K, (Table 1 and Figure 5) shows a monotonic decrease as the RuO2 content is increased and TIR increases from 0.12 to 0.66. The thermopower of binary calcium cobaltite (CC-1) is 151 μV/K [28] and
Figure 4. Seebeck Coefficient of ternary CaCo1−xRuxOy samples as a function of temperature showing that increase in S temperature decreases with increasing Ru content.
Figure 5. Thermopower, at 600 K, of CaCo1−xRuxOy samples as a function of TIR with S decreasing with TIR.
that of binary calcium ruthenate (CaR-5) is 37 μV/K [27]. As cobalt ions are progressively replaced by ruthenium to form the CaCo1−xRuxOy series, thermopower is observed to decrease monotonically. However if the thermopower of CC-1 and CCR-6 are compared it was observed that the thermopower increases from 151 to 187 μV/K. Unlike the trend observed in resistivity, the thermopower of CaCo1−xRuxOy system exhibits a maximum, even though any variation in resistivity is reflected in a similar variation in thermopower in most semiconductors. However, if only the CaCo1−xRuxOy (ternary) compositions are considered, the resistivity and Seebeck coefficient vary as expected in a semiconducting system. For the CaCo1−xRuxOy compositions (Table 1) while the resistivity decreases from 8.24 × 10−4 to 1.42 × 10−4 Ω.m, the Seebeck coefficient also decreases from 187 to 35 μV/K in the same composition range. Comparing the Seebeck coefficients of CCR-4 and -6, it is observed that replacement of Ca by Ru ions reduces the Seebeck coefficient by a factor of 1.5. Similarly comparing the Seebeck coefficients of CCR-2 and -3, it was observed that replacement of Co by Ca also reduces the Seebeck coefficient, but not significantly.
The two samples CCR-1 and CCR-2 have very similar Seebeck coefficients 38 and 35 μV/K respectively, while the binary calcium ruthenate (CaR-5) has a thermopower of 37 μV/K. This behavior of thermopower is similar to that of resistivity discussed earlier for these two samples. This insensitivity of thermopower to RuO2 concentration in the calcium ruthenate samples agrees with previously reported data [27,30]. The invariance of thermopower signifies that the electronic structure and especially the density of states of these ceramics (e.g. SrRuO3 and CaRuO3) are unaffected not only by the various substitutions, but also by the type of the other cations (apart from Ru) constituting the ceramic. The Seebeck coefficient of the other two samples, CCR-5 and -6 varies with compositionfrom 127 to 187 µV/K.
The power factor, S2/ρ, (or S2σ, where σ is the conductivity) of the four CaCo1−xRuxOy compositions (CCR-1, 2, 5 and 6) is depicted as a function of temperature in Figure 6. The samples with TIR < 0.5 exhibit an increase in the power factor as the temperature is increased from 300 to 600 K, while the samples with TIR ≥ 0.5 also show an increase in power factor but not to the same extent as the earlier group. The power factor of the CaCo1–xRuxOy samples at 600 K varies within a rather small range [Figure 7]: 8.6 × 10−6 to 4.3 × 10−5 Wm−1K−2. The combined effect of S and ρ on the power factor as brought about by compositional changes is listed in Table 1 and Figure 7. Compared to the data available in the literature for substitution of Co in calcium cobaltites by other transition metal ions, such as Fe, Ni, Mn etc., this reduction in the power factor of CaCo1−xRuxOy compositions is not meager. Comparing the power factors of the binary calcium cobaltite (CC-1) and the CaCo1−xRuxOy sample with the highest Ru concentration (CCR-1, TIR of 0.66) it was observed that the power factor diminishes by a factor of 2.8. This decrease is higher than the observation of Yao et al., [24] who observed a reduction of power factor by a factor of only 0.5 to 1.1 when Fe, Ni, Mn or Cu was substituted for Co. Even though this decrease is not substantially higher compared to their result, it could be due to the fact that Ru is a 4d transition metal compared to the 3d transition
Figure 6. Powerfactor of CaCo1−xRuxOy samples as a function of temperature with powerfactor decreasing with temperature for all the samples.
Figure 7. Powerfactor, at 600 K, of CaCo1−xRuxOy samples as a function of TIR shows that change in powerfactor is less than an order of magnitude.
metals, Fe, Ni, Mn and Cu, that Yao et al., used. As a result of the decrease in the thermopower (Figure 6) caused by Ru-substitution of Co ions, the power factor (S2/ρ) is found to be reduced, though only by less than an order of magnitude, diminishing its value as a prospective thermoelectric material. However, its value as an electrode material is significantly enhanced.
Scanning electron microscopy/EDS analyses performed on the CaCo1−xRuxOy samples reveal that substitution of Ru for Co in the range of compositions (Table 1) does not lead to the formation of a significant second phase. However, a minority phase (~1% - 2%) has been observed in most samples. The small size of this phase (~1 micron) renders it difficult to precisely determine its composition. Apparently, Ru ions have replaced Co in the major phase or vice versa depending on the composition (Figure 8(a) and Figure 9(a)). However, depending on the composition, two distinctly different microstructures have been observed. The majority phase assumes a needle shaped structure, for compositions having TIR < 0.5, such as those observed in samples CCR-5 and CCR-6 (Figure 8(a)). This microstructure is not very different from that of CC-1, the binary calcium cobaltite, as can be observed from Figure 8(b). These compositions (CCR-5 and -6) exhibit higher resistivities as well as higher Seebeck coefficients (Table 1, Figures 4-7) compared to the compositions with TIR ≥ 0.5. For CCR-1 and -2 which have a TIR ≥ 0.5, the microstructure contains plate-like crystals as presented in Figure 9(a) (CCR-2). Binary calcium ruthenate (CaR-5), in which the only phase is CaRuO3, was also demonstrated [27] to have a similar plate-like microstructure, as depicted in Figure 9(b). Compositions with TIR ≥ 0.5 (CCR-1 & -2) exhibit similar (lower) resistivities and Seebeck coefficients.
X-ray diffraction patterns of the four CaCo1–xRuxOy samples (CCR-1, 2, 5 and 6) are provided in Figures 10 and 11 along with that of CaR-5 and CC-1 to study the evolution of the phases with increasing TIR. CCR-1 and -2, which have a TIR ≥ 0.5, have CaRuO3 as the major phase with CoO and CaO being the minor phases while CaR-5, the binary calcium ruthenate, has only the CaRuO3 phase as can be observed from Figure 10. The XRD patterns of CCR-6 and -5, which have a TIR < 0.5, exhibit the predominance of the Ca9Co12O28 phase with minor amounts of Ca3Co2O6(CCR-6) or CaRuO3 (CCR-5) along with CaO as seen from Figure 11. Ca3Co2O6 and Ca2Co2O5 are the major and minor phases respectively in the CC-1 composition as shown in Figure 11.
Based on the above, it can be proposed that the CaCo1−xRuxOy samples on either side of the central line (TIR = 0.5) have similar microstructures, phase assemblage, resistivities and Seebeck coefficients.
Ru has been identified as a unique dopant [29] in ceramic compounds containing transition ion oxides because the electronic structure of Ru, similar to that of the 3d transition metal ions (TI), favors the interactions between the TI and Ru and improves metallicity. This metallicity enhancement with Ru addition has been reported [29] in CaMnO3 where the ferromagnetic properties of RuO2 seem to contribute to conductivity enhancement as compared to non-magnetic cations such as Nb and Mo. It was concluded that when CaMnO3 was doped with Ru, ferromagnetic interactions and thus metallicity were en-
(a)
(b)
Figure 8. (a) SEM micrograph of the CCR-6 sample, which exhibits microstructure and transport properties similar to the binary calcium cobaltite (CC-1) shown in (b) SEM micrograph of the microstructure of CC-1 sample (binary calcium cobaltite), showing acicular structure.
hanced because of superexchange interactions between Mn and Ru and, furthermore, the eg electron of Ru4+ actively participated in the transport phenomena. As the 4d orbitals of Ru ions are more extended than its 3d counterparts, enhanced orbital overlapping (with TI 3-d and oxygen 2-p orbitals) takes place, resulting in higher conductivity. The effect of equimolar substitution of cobalt ions by ruthenium on the thermopower also proves the enhanced metallic properties of the ceramic. As a result of this increasing metallicity of the ceramic, the thermopower decreases monotonically.
The effect of Ru-substitution on the thermoelectric properties of CaCo1−xRuxOy samples has been found to be similar to those observed earlier [24] in Ca3Co4−xCuxO9+δ, where Cu substitution for Co ions resulted in the decrease of electrical resistivity and themopower. It was demonstrated that substitution of Cu for Co took place in
(a)
(b)
Figure 9. (a) Microstructure of the CCR-2 sample which has calcium ruthenate as the major phase and has microstructure and transport properties similar to the binary calcium ruthenate shown in (b) Microstructure of CaR-5 [27] showing plate like structure of binary calcium ruthenate.
the Ca2CoO3 layer, while substitution by Fe, Ni or Mn took place in CoO2 layer [24]. Since the CoO2 layer is the conducting path in these cobaltites, such a “defect” would present itself as a scattering center for the carriers as it would block the passage of the latter, thereby increasing the resistivity and the activation energy for conduction. However, a substitution (Cu for Co) in the Ca2CoO3 layers would not disturb the conduction path and the activation energy would remain constant or was even found to be lowered. In Ca3Co4O9+d compounds, the effective carriers are holes, as evidenced from the positive thermopower. Usually, the Co ions are trivalent or tetravalent in the Ca2CoO3 layers and the Cu ion is divalent in CuO. The substitution of Cu for the Co in the Ca2CoO3 layer increases the hole concentration and leads to an increase in conductivity [24]. In the case of Ru, an
Figure 10. XRD patterns of CCR-1, -2 and CaR-5; showing that CaRuO3 is the major phase with CaO and CoO as minor phases.
Figure 11. XRD patterns of CCR-5, -6 and CC-1; with Ca9Co12O28 being the major phase in CaCo1−xRuxOy samples and Ca3Ca2O6 in CC-1.
increase in the hole concentration is ruled out because the valence state of Ru cannot be less than that of Co. As Ru is tetravalent, the increased conductivity cannot be ascribed to increase in carrier concentration. Hence the same explanation, obviously, does not hold.
Recently, it has been demonstrated by Tran and others [31], using x-ray photoemission spectroscopy and a cluster model calculation, that considerable hybridization of the Cu 3d and Ru 4d orbitals exist in CaCu3Ru4O12 and render the Ru 4d electrons highly itinerant. In our system, it was found that equimolar substitution of Co (3d) by Ru (4d) reduces the resistivity as well as the thermopower of the system. This reduction in resistivity and thermopower supports the contention of Tran et al., [31] and indicates that Co 3d and Ru 4d orbitals do overlap and render the Ru-substituted CaCo1−xRuxOy samples more conductive (metallic). Thus the decrease in resistivity and thermopower may be ascribed to the superior overlapping of the O 2p, Co 3d and Ru 4d orbitals as compared to the binary calcium cobaltite system facilitating transport of the carriers.
It was observed that when Co was substituted by various 3d transition metals such as Fe, Ni, Mn or Cu (to the extent of 0.6 moles), the maximum power factor variation was 0.5 times the value before substitution [24]. In the present investigation where Ru was substituted for Co (to the extent of only 0.33 moles), the power factor variation observed is from 2 (up) to 2.4 (down) times the value observed before substitution. As the 4d orbitals of Ru ions are more extended than its 3d counterparts, like that of Fe, Ni, Mn or Cu, enhanced orbital overlapping (with Co 3d and oxygen 2p orbitals) takes place, resulting in higher conductivity. This enhanced orbital overlapping also reduces the band gap, resulting in the reduction of thermopower [32]. Thus increased metallicity due to enhanced orbital overlapping explains the higher reduction/increase in the power factor of Ru substituted calcium cobaltite samples compared to the other 3d transition metal, such as Fe, Ni, Mn or Cu, substituted calcium cobaltite samples.
4. Conclusion
Effective substitution of Co by Ru ions in the calciumcobalt-ruthenium oxide system (CCR), represented by the general formula CaCo1−xRuxOy, with x varying from 0.06 to 0.33, has been demonstrated without the formation of a substantial second phase. Both thermopower and resistivity of the CaCo1−xRuxOy samples decrease sharply until a TIR, (Ru/Co + Ru ratio), of 0.5 is reached, beyond which they are insensitive to the TIR. The Seebeck coefficient varies from 187 µV/K, at a TIR of 0.12, to 35 µV/K, at a TIR of 0.66, while the resistivity decreases from 8.2 × 10−4 to 1.4 × 10−4 Ω∙m in the same TIR range. The decrease in thermopower and resistivity is suggested to be caused by efficient orbital overlapping of the 4d Ru orbitals with Co 3d and oxygen 2p orbitals. Based on the transport properties, the members of the CaCo1−xRuxOy series having higher TIR (≥0.5) are found to behave similar to the calcium ruthenates, i.e. relative insensitivity of transport properties to compositional variation and also have microstructure, electrical resistivity and thermopower values similar to that of the binary CaR-5. The members of CaCo1−xRuxOy series with TIR < 0.5 have a different microstructure, higher resistivity and higher thermopower compared to the members with TIR > 0.5, but similar to that of the binary CC-1 composition. The power factor, however, does not change appreciably with TIR, changing by less than an order of magnitude. These ternary CaCo1−xRuxOy samples may be used for applications where lower resistivity is required, like electrode materials.
Acknowledgements
We gratefully acknowledge the contributions of Dr. Igor Vidensky, Ms. NiveenFahmy, Dr. Thomas Barnard and Wei Zhou in sample preparation.
REFERENCES
- B. Raveau and A. Maignan, “The Extraordinry Properties of Magnetic Oxides,” Europhysics News, Vol. 34, No. 6, 2003, pp. 238-240. http://dx.doi.org/10.1051/epn:2003612
- C. Frontera, J. L. Garcıa-Munoz, A. Llobet and M. A. G. Aranda, “Selective Spin-Stateswitch and Metal-Insulator Transition in GdBaCo2O5.5,” Physical Review B, Vo. 65, 2002, Article ID: 180405. http://dx.doi.org/10.1103/PhysRevB.65.180405
- B. C. Zhao, Y. P. Sun and W. H. Song, “Magnetic and Transport Properties in the Ti Doped Cobaltite Ca3Co4−xTixO9 (0≤x≤0.8)≤Single Crystals,” Journal of Applied Physics, Vol. 99, 2006, Article ID: 073906. http://dx.doi.org/10.1063/1.2190027
- A. C. Masset, C. Michel, A. Maignan, M. Hervieu, O. Toulemonde, F. Studer, B. Raveau and J. Hejtmanek, “Misfit-Layered Cobaltite with an Anisotropic Giant Magnetoresistance:Ca3Co4O9,” Physical Review B, Vol. 62, No. 1, 2000, pp. 166-175. http://dx.doi.org/10.1103/PhysRevB.62.166
- Y. Miyazaki, K. Kudo, M. Akoshima, Y. Ono, Y. Koike and T. Kajitani, “Low-Temperature Thermoelectric Properties of the Composite Crystal [Ca 2CoO 3.34] 0.614[CoO 2],” Japanese Journal of Applied Physics, Vol. 39, No. 6A, 2000, pp. L531-L533. http://dx.doi.org/10.1143/JJAP.39.L531
- S. Lambert, H. Leligny and D. Grebille, “Three Forms of the Misfit Layered Cobaltite [Ca2CoO3] [CoO2]1.62·A 4D Structural Investigation,” Journal of Solid State Chemistry, Vol. 160, No. 2, 2001, pp. 322-331. http://dx.doi.org/10.1006/jssc.2001.9235
- Y. Miyazaki, M. Onoda, T. Oku, M. Kikuchi, Y. Ishii, Y. Ono, Y. Morii and T. Kajitani, “Modulated Structure of the Thermoelectric Compound [Ca2CoO3]0.62CoO2,” Journal of the Physical Society of Japan, Vol. 71, No. 2, 2002, pp. 491-497. http://dx.doi.org/10.1143/JPSJ.71.491
- Marc Respaud, Carlos Frontera, Jose Luis Garcıa-Munoz, Miguel Angel G. Aranda, Bertrand Raquet, Jean Marc Broto, HarisonRakoto, Michel Goiran, Anna Llobet and Juan Rodrıguez-Carvajal, “Magnetic and Magnetotransport Properties of GdBaCo2O5+δA High Magnetic-Field Study,” Physical Review B, Vol. 64, 2001, Article ID: 214401. http://dx.doi.org/10.1103/PhysRevB.64.214401
- A. Maignan, C. Martin, D. Pelloquin, N. Nguyen, B. Raveau,“Structural and Magnetic Studies of Ordered Oxymgen-Deficient PerovskitesLnBaCo2O5+δ, Closely Related to the ‘112’ Structure,” Journal of Solid State Chemistry, Vol. 142, No. 2, 1999, pp. 247-260. http://dx.doi.org/10.1006/jssc.1998.7934
- G. Cao, S. McCall, M. Shepard, J. E. Crow and R. P. Guertin, “Magnetic and Transport Properties of SingleCrystal Ca2RuO4: Relationship to Superconducting Sr2RuO4,” Physical Review B, Vol. 56, No. 6, 1997, pp. R2916- R2919. http://dx.doi.org/10.1103/PhysRevB.56.R2916
- S. Nakatsuji, S. I. Ikeda and Y. Maeno, “Ca 2RuO 4: New Mott Insulators of Layered Ruthenate,” Journal of the Physical Society of Japan, Vol. 66, No. 7, 1997, pp. 1868- 1871. http://dx.doi.org/10.1143/JPSJ.66.1868
- A. Callaghan, W. Moller and R. Ward, “Magnetic Interactions in Ternary Ruthenium Oxides,” Inorganic Chemistry, Vol. 5, No. 9, 1966, pp. 1572-1576. http://dx.doi.org/10.1021/ic50043a023
- M.K. Crawford, R.L. Harlow, W. Marshall, Z. Li, G. Cao, R. L. Lindstrom, Q. Huang and J. W. Lynn, “Structure and Magnetism of Single Crystal Sr4Ru3O10A Ferromagnetic Triple-Layer Ruthenate,” Physical Review B, Vol. 65, 2002, Article ID: 214412. http://dx.doi.org/10.1103/PhysRevB.65.214412
- Y. Maeno, H. Hashimoto Y. Maeno, H. Hashimoto, K. Yoshida, S. Nishizaki, T. Fujita, J. G. Bednorz and F. Lichtenberg, “Superconductivity in a Layered Perovskite without Copper,” Nature, Vol. 372, 1994, pp. 532-534. http://dx.doi.org/10.1038/372532a0
- G. Cao, S. McCall, J. E. Crow and R. P. Guertin, “Observation of a Metallic Antiferromagnetic Phase and Metal to Nonmetal Transition in Ca3Ru2O7,” Physical Review Letter, Vol. 78, No. 9, 1997, pp. 1751-1754. http://dx.doi.org/10.1103/PhysRevLett.78.1751
- C.B. Eom, R.J. Cava , R. M. Fleming, Julia M. Phillips, R. B. vanDover, J. H. Marshall, J. W. P. Hsu, J. J. Krajewski and W. F. Peck Jr., “Single-Crystal Epitaxial Thin Films of the Isotropic Metallic Oxides Sr1–xCaxRuO3 (0 ≤ x ≤ 1),” Science, Vol. 258, No. 5089, 1992, pp. 1766-1769. http://dx.doi.org/10.1126/science.258.5089.1766
- X. Wang, Y. Xin, P. A. Stampe and R. J. Kennedy, “Epitaxial thin film growth of Ca2RuO4+ Dby Pulsed Laser Deposition,” Applied Physics Letters, Vol. 85, No. 25, 2004, p. 6146. http://dx.doi.org/10.1063/1.1841451
- S. Rane, M. Prudenziati and B. Morten, “CaRuO3-Based ‘Green’ Thick Film Resistors,” Journal of Active and Passive Electronic Devices. Vol. 123, 2005.
- T. C. Gibb, R. G. Greatrex, N. N. Greenwood and P. Kaspi, “Ruthenium-99 Mössbauer Studies of the Magnetic Properties of Ternary and Quaternary Ruthenium(IV) Oxides,” Journal of the Chemical Society, Dalton Transactions, 1973, p. 1253.
- L. Klein, L. Antognazza, T. H. Geballe, M. R. Beasley and A. Kapitulnik, “Possible Non-Fermi-Liquid Behavior of CaRuO3,” Physical Review B, Vol. 60, No. 3, 1999, pp. 1448-1451. http://dx.doi.org/10.1103/PhysRevB.60.1448
- I. Felner, I. Nowik, I. M. Bradaric and M. Gospodinov, CaRuO3 Is Not a Paramagnetic Material,” Physical Review B, Vol. 62, 2000, pp. 11332-11335. http://dx.doi.org/10.1103/PhysRevB.62.11332
- O. Friedt, M. Braden, G. André, P. Adelmann, S. Nakatsuji and Y. Maeno, “Structural and Magnetic Aspects of the Metal-Insulator Transition in Ca2-xSrxRuO4,” Physical Review B, Vol. 63, 2001, Article ID: 174432. http://dx.doi.org/10.1103/PhysRevB.63.174432
- P. A. Cox, R.G. Egdell, J. B. Goodenough, A. Hamnett and C. CNaish, “The Metal-to-Semiconductor Transition in Ternary Ruthenium (IV) Oxides: A Study by Electron Spectroscopy,” Journal of Physics C: Solid State Physics, Vol. 16, No. 32, 1983, p. 6221. http://dx.doi.org/10.1088/0022-3719/16/32/014
- Q. Yao, D. L. Wang, L. D. Chen, X. Shi, and M. Zhou, “Effects of Partial Substitution of Transition Metals for Cobalt on the High-Temperature Thermoelectric Properties of Ca3Co4O9+δ,” Journal of Applied Physics, Vol. 97, No. 10, 2005, Article ID: 103905. http://dx.doi.org/10.1063/1.1898443
- I. Matsubara, R. Funahashi, T. Takeuchi and S. Sodeoka, “Thermoelectric Properties of Spark Plasma Sintered Ca2.75Gd0.25Co4O9 Ceramics,” Journal of Applied Physics, Vol. 90, No. 1, 2001, p. 462. http://dx.doi.org/10.1063/1.1378056
- L. J. Van der Pauw, “A Method of Measuring Specific Resistivity and HALL Effect of Discs of Arbitrary Shape,” Philips Research Reports, Vol. 13, 1958, pp. 1-9.
- S. Annamalai, I. Vidensky, I. L. Pegg and B. Dutta, “Effect of Cation Stoichiometry on the Transport Properties of Calcium Ruthenium Oxide Ceramics,” Journal of Materials Science, Vol. 43, No. 14, 2008. pp. 4996-5004. http://dx.doi.org/10.1007/s10853-008-2739-2
- S. Annamalai, R. P. Bhatta, I. L. Pegg and B. Dutta, “The effect of Stoichiometry on the Thermoelectric Properties of Calcium Cobaltite,” Energy Materials Proceedings, MS&T09 Conference, Pittsburgh, October 2009, pp 229- 240.
- S. Hébert, C. Martin, A. Maignan, R. Fresard, J. Hejtmanek and B. Raveau, “Large Thermopower in Metallic Oxides: Misfitcobaltites and mangano-ruthenates,” Proceedings of the 6th European Workshop on Thermoelectrics, Freiburg, 2001.
- Y. Klein, S. Hébert, A. Maignan, S. Kolesnik, T. Maxwell and B. Dabrows, “Insensitivity of the Band Structure of Substituted SrRuO3 as Probed by Seebeck Coefficient Measurements,” Physical Review B, Vol. 73, No. 5, 2006, Article ID: 052412. http://dx.doi.org/10.1103/PhysRevB.73.052412
- T. T. Tran, K. Takubo, T. Mizokawa, W. Kobayashi and I. Terasaki, “Electronic Structure of CaCu3Ru4O12 Studied by X-Ray Photoemission Spectroscopy,” Physical Review B, Vol. 73, 2006, Article ID: 193105. http://dx.doi.org/10.1103/PhysRevB.73.193105
- G. S. Nolas, J. Sharp and H. J. Goldsmid, “Thermoelectrics: Basic Principles and New Materials Development,” Springer, Berlin, 2001.
NOTES
*Corresponding author.