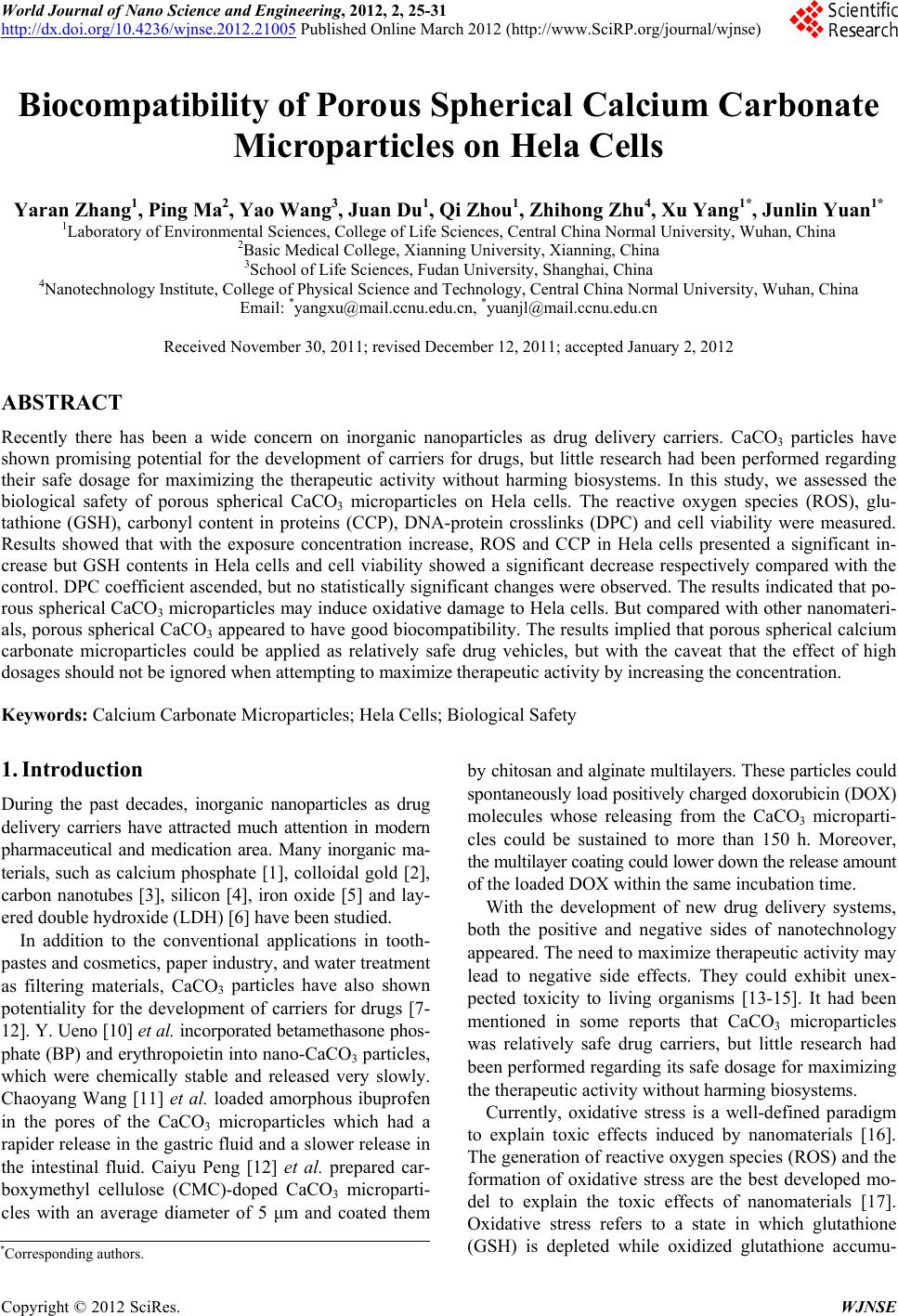 World Journal of Nano Science and Engineering, 2012, 2, 25-31 http://dx.doi.org/10.4236/wjnse.2012.21005 Published Online March 2012 (http://www.SciRP.org/journal/wjnse) 25 Biocompatibility of Porous Spherical Calcium Carbonate Microparticles on Hela Cells Yaran Zhang1, Ping Ma2, Yao Wang3, Juan Du1, Qi Zhou1, Zhihong Zhu4, Xu Yang1*, Junlin Yuan1* 1Laboratory of Environmental Sciences, College of Life Sciences, Central China Normal University, Wuhan, China 2Basic Medical College, Xianning University, Xianning, China 3School of Life Sciences, Fudan University, Shanghai, China 4Nanotechnology Institute, College of Physical Science and Technology, Central China Normal University, Wuhan, China Email: *yangxu@mail.ccnu.edu.cn, *yuanjl@mail.ccnu.edu.cn Received November 30, 2011; revised December 12, 2011; accepted January 2, 2012 ABSTRACT Recently there has been a wide concern on inorganic nanoparticles as drug delivery carriers. CaCO3 particles have shown promising potential for the development of carriers for drugs, but little research had been performed regarding their safe dosage for maximizing the therapeutic activity without harming biosystems. In this study, we assessed the biological safety of porous spherical CaCO3 microparticles on Hela cells. The reactive oxygen species (ROS), glu- tathione (GSH), carbonyl content in proteins (CCP), DNA-protein crosslinks (DPC) and cell viability were measured. Results showed that with the exposure concentration increase, ROS and CCP in Hela cells presented a significant in- crease but GSH contents in Hela cells and cell viability showed a significant decrease respectively compared with the control. DPC coefficient ascended, but no statistically significant changes were observed. The results indicated that po- rous spherical CaCO3 microparticles may induce oxidative damage to Hela cells. But compared with other nanomateri- als, porous spherical CaCO3 appeared to have good biocompatibility. The results implied that porous spherical calcium carbonate microparticles could be applied as relatively safe drug vehicles, but with the caveat that the effect of high dosages should not be ignored when attempting to maximize therapeutic activity by increasing the concentration. Keywords: Calcium Carbonate Microparticles; Hela Cells; Biological Safety 1. Introduction During the past decades, inorganic nanoparticles as drug delivery carriers have attracted much attention in modern pharmaceutical and medication area. Many inorganic ma- terials, such as calcium phosphate [1], colloidal gold [2], carbon nanotubes [3], silicon [4], iron oxide [5] and lay- ered double hydroxide (LDH) [6] have been studied. In addition to the conventional applications in tooth- pastes and cosmetics, paper industry, and water treatment as filtering materials, CaCO3 particles have also shown potentiality for the development of carriers for drugs [7- 12]. Y. Ueno [10] et al. incorporated betamethasone phos- phate (BP) and erythropoietin into nano-CaCO3 particles, which were chemically stable and released very slowly. Chaoyang Wang [11] et al. loaded amorphous ibuprofen in the pores of the CaCO3 microparticles which had a rapider release in the gastric fluid and a slower release in the intestinal fluid. Caiyu Peng [12] et al. prepared car- boxymethyl cellulose (CMC)-doped CaCO3 microparti- cles with an average diameter of 5 μm and coated them by chitosan and alginate multilayers. These particles could spontaneously load positively charged doxorubicin (DOX) molecules whose releasing from the CaCO3 microparti- cles could be sustained to more than 150 h. Moreover, the multilayer coating could lower down the release amount of the loaded DOX within the same incubation time. With the development of new drug delivery systems, both the positive and negative sides of nanotechnology appeared. The need to maximize therapeutic activity may lead to negative side effects. They could exhibit unex- pected toxicity to living organisms [13-15]. It had been mentioned in some reports that CaCO3 microparticles was relatively safe drug carriers, but little research had been performed regarding its safe dosage for maximizing the therapeutic activity without harming biosystems. Currently, oxidative stress is a well-defined paradigm to explain toxic effects induced by nanomaterials [16]. The generation of reactive oxygen species (ROS) and the formation of oxidative stress are the best developed mo- del to explain the toxic effects of nanomaterials [17]. Oxidative stress refers to a state in which glutathione (GSH) is depleted while oxidized glutathione accumu- *Corresponding authors. C opyright © 2012 SciRes. WJNSE
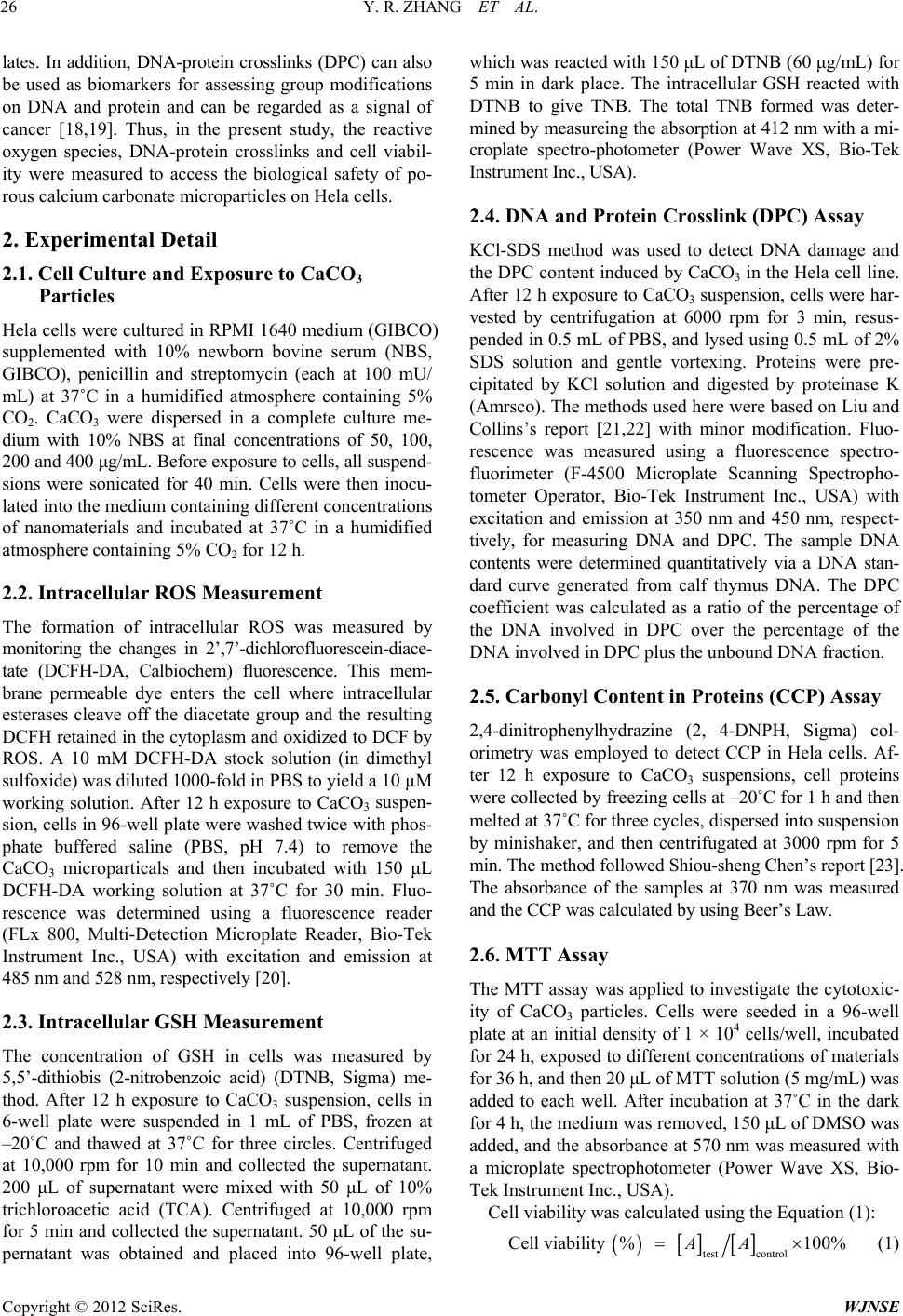 Y. R. ZHANG ET AL. 26 lates. In addition, DNA-protein crosslinks (DPC) can also be used as biomarkers for assessing group modifications on DNA and protein and can be regarded as a signal of cancer [18,19]. Thus, in the present study, the reactive oxygen species, DNA-protein crosslinks and cell viabil- ity were measured to access the biological safety of po- rous calcium carbonate microparticles on Hela cells. 2. Experimental Detail 2.1. Cell Culture and Exposure to CaCO3 Particles Hela cells were cultured in RPMI 1640 medium (GIBCO) supplemented with 10% newborn bovine serum (NBS, GIBCO), penicillin and streptomycin (each at 100 mU/ mL) at 37˚C in a humidified atmosphere containing 5% CO2. CaCO3 were dispersed in a complete culture me- dium with 10% NBS at final concentrations of 50, 100, 200 and 400 μg/mL. Before exposure to cells, all suspend- sions were sonicated for 40 min. Cells were then inocu- lated into the medium containing different concentrations of nanomaterials and incubated at 37˚C in a humidified atmosphere containing 5% CO2 for 12 h. 2.2. Intracellular ROS Measurement The formation of intracellular ROS was measured by monitoring the changes in 2’,7’-dichlorofluorescein-diace- tate (DCFH-DA, Calbiochem) fluorescence. This mem- brane permeable dye enters the cell where intracellular esterases cleave off the diacetate group and the resulting DCFH retained in the cytoplasm and oxidized to DCF by ROS. A 10 mM DCFH-DA stock solution (in dimethyl sulfoxide) was diluted 1000-fold in PBS to yield a 10 µM working solution. After 12 h exposure to CaCO3 suspen- sion, cells in 96-well plate were washed twice with phos- phate buffered saline (PBS, pH 7.4) to remove the CaCO3 microparticals and then incubated with 150 μL DCFH-DA working solution at 37˚C for 30 min. Fluo- rescence was determined using a fluorescence reader (FLx 800, Multi-Detection Microplate Reader, Bio-Tek Instrument Inc., USA) with excitation and emission at 485 nm and 528 nm, respectively [20]. 2.3. Intracellular GSH Measurement The concentration of GSH in cells was measured by 5,5’-dithiobis (2-nitrobenzoic acid) (DTNB, Sigma) me- thod. After 12 h exposure to CaCO3 suspension, cells in 6-well plate were suspended in 1 mL of PBS, frozen at –20˚C and thawed at 37˚C for three circles. Centrifuged at 10,000 rpm for 10 min and collected the supernatant. 200 μL of supernatant were mixed with 50 μL of 10% trichloroacetic acid (TCA). Centrifuged at 10,000 rpm for 5 min and collected the supernatant. 50 μL of the su- pernatant was obtained and placed into 96-well plate, which was reacted with 150 μL of DTNB (60 μg/mL) for 5 min in dark place. The intracellular GSH reacted with DTNB to give TNB. The total TNB formed was deter- mined by measureing the absorption at 412 nm with a mi- croplate spectro-photometer (Power Wave XS, Bio-Tek Instrument Inc., USA). 2.4. DNA and Protein Crosslink (DPC) Assay KCl-SDS method was used to detect DNA damage and the DPC content induced by CaCO3 in the Hela cell line. After 12 h exposure to CaCO3 suspension, cells were har- vested by centrifugation at 6000 rpm for 3 min, resus- pended in 0.5 mL of PBS, and lysed using 0.5 mL of 2% SDS solution and gentle vortexing. Proteins were pre- cipitated by KCl solution and digested by proteinase K (Amrsco). The methods used here were based on Liu and Collins’s report [21,22] with minor modification. Fluo- rescence was measured using a fluorescence spectro- fluorimeter (F-4500 Microplate Scanning Spectropho- tometer Operator, Bio-Tek Instrument Inc., USA) with excitation and emission at 350 nm and 450 nm, respect- tively, for measuring DNA and DPC. The sample DNA contents were determined quantitatively via a DNA stan- dard curve generated from calf thymus DNA. The DPC coefficient was calculated as a ratio of the percentage of the DNA involved in DPC over the percentage of the DNA involved in DPC plus the unbound DNA fraction. 2.5. Carbonyl Content in Proteins (CCP) Assay 2,4-dinitrophenylhydrazine (2, 4-DNPH, Sigma) col- orimetry was employed to detect CCP in Hela cells. Af- ter 12 h exposure to CaCO3 suspensions, cell proteins were collected by freezing cells at –20˚C for 1 h and then melted at 37˚C for three cycles, dispersed into suspension by minishaker, and then centrifugated at 3000 rpm for 5 min. The method followed Shiou-sheng Chen’s report [23]. The absorbance of the samples at 370 nm was measured and the CCP was calculated by using Beer’s Law. 2.6. MTT Assay The MTT assay was applied to investigate the cytotoxic- ity of CaCO3 particles. Cells were seeded in a 96-well plate at an initial density of 1 × 104 cells/well, incubated for 24 h, exposed to different concentrations of materials for 36 h, and then 20 μL of MTT solution (5 mg/mL) was added to each well. After incubation at 37˚C in the dark for 4 h, the medium was removed, 150 μL of DMSO was added, and the absorbance at 570 nm was measured with a microplate spectrophotometer (Power Wave XS, Bio- Tek Instrument Inc., USA). Cell viability was calculated using the Equation (1): test control Cell viability % 100%AA (1) Copyright © 2012 SciRes. WJNSE
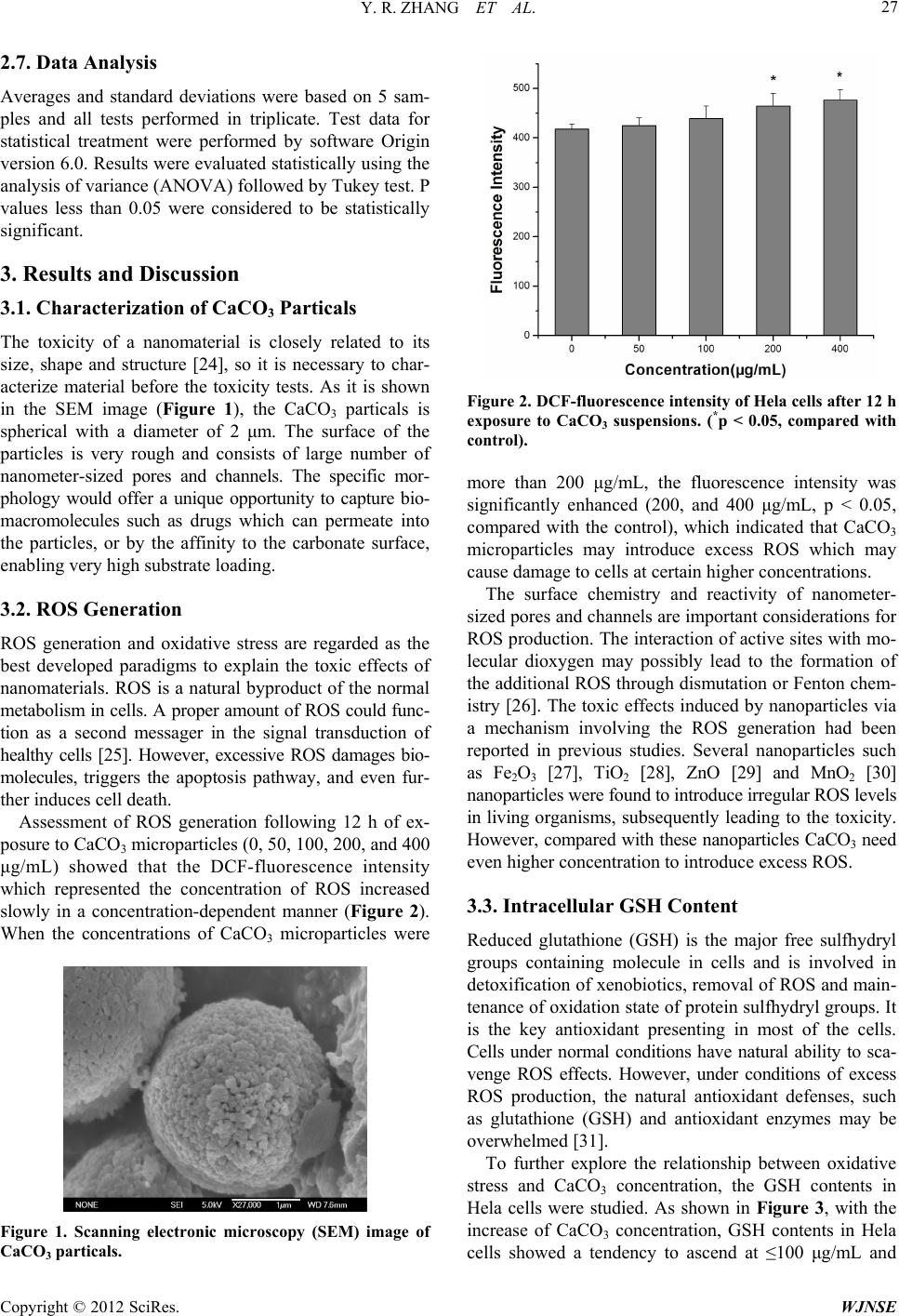 Y. R. ZHANG ET AL. 27 2.7. Data Analysis Averages and standard deviations were based on 5 sam- ples and all tests performed in triplicate. Test data for statistical treatment were performed by software Origin version 6.0. Results were evaluated statistically using the analysis of variance (ANOVA) followed by Tukey test. P values less than 0.05 were considered to be statistically significant. 3. Results and Discussion 3.1. Characterization of CaCO3 Particals The toxicity of a nanomaterial is closely related to its size, shape and structure [24], so it is necessary to char- acterize material before the toxicity tests. As it is shown in the SEM image (Figure 1), the CaCO3 particals is spherical with a diameter of 2 μm. The surface of the particles is very rough and consists of large number of nanometer-sized pores and channels. The specific mor- phology would offer a unique opportunity to capture bio- macromolecules such as drugs which can permeate into the particles, or by the affinity to the carbonate surface, enabling very high substrate loading. 3.2. ROS Generation ROS generation and oxidative stress are regarded as the best developed paradigms to explain the toxic effects of nanomaterials. ROS is a natural byproduct of the normal metabolism in cells. A proper amount of ROS could func- tion as a second messager in the signal transduction of healthy cells [25]. However, excessive ROS damages bio- molecules, triggers the apoptosis pathway, and even fur- ther induces cell death. Assessment of ROS generation following 12 h of ex- posure to CaCO3 microparticles (0, 50, 100, 200, and 400 μg/mL) showed that the DCF-fluorescence intensity which represented the concentration of ROS increased slowly in a concentration-dependent manner (Figure 2). When the concentrations of CaCO3 microparticles were Figure 1. Scanning electronic microscopy (SEM) image of CaCO3 particals. Figure 2. DCF-fluorescence intensity of Hela cells after 12 h exposure to CaCO3 suspensions. (*p < 0.05, compared with control). more than 200 μg/mL, the fluorescence intensity was significantly enhanced (200, and 400 μg/mL, p < 0.05, compared with the control), which indicated that CaCO3 microparticles may introduce excess ROS which may cause damage to cells at certain higher concentrations. The surface chemistry and reactivity of nanometer- sized pores and channels are important considerations for ROS production. The interaction of active sites with mo- lecular dioxygen may possibly lead to the formation of the additional ROS through dismutation or Fenton chem- istry [26]. The toxic effects induced by nanoparticles via a mechanism involving the ROS generation had been reported in previous studies. Several nanoparticles such as Fe2O3 [27], TiO2 [28], ZnO [29] and MnO2 [30] nanoparticles were found to introduce irregular ROS levels in living organisms, subsequently leading to the toxicity. However, compared with these nanoparticles CaCO3 need even higher concentration to introduce excess ROS. 3.3. Intracellular GSH Content Reduced glutathione (GSH) is the major free sulfhydryl groups containing molecule in cells and is involved in detoxification of xenobiotics, removal of ROS and main- tenance of oxidation state of protein sulfhydryl groups. It is the key antioxidant presenting in most of the cells. Cells under normal conditions have natural ability to sca- venge ROS effects. However, under conditions of excess ROS production, the natural antioxidant defenses, such as glutathione (GSH) and antioxidant enzymes may be overwhelmed [31]. To further explore the relationship between oxidative stress and CaCO3 concentration, the GSH contents in Hela cells were studied. As shown in Figure 3, with the increase of CaCO3 concentration, GSH contents in Hela cells showed a tendency to ascend at ≤100 μg/mL and Copyright © 2012 SciRes. WJNSE
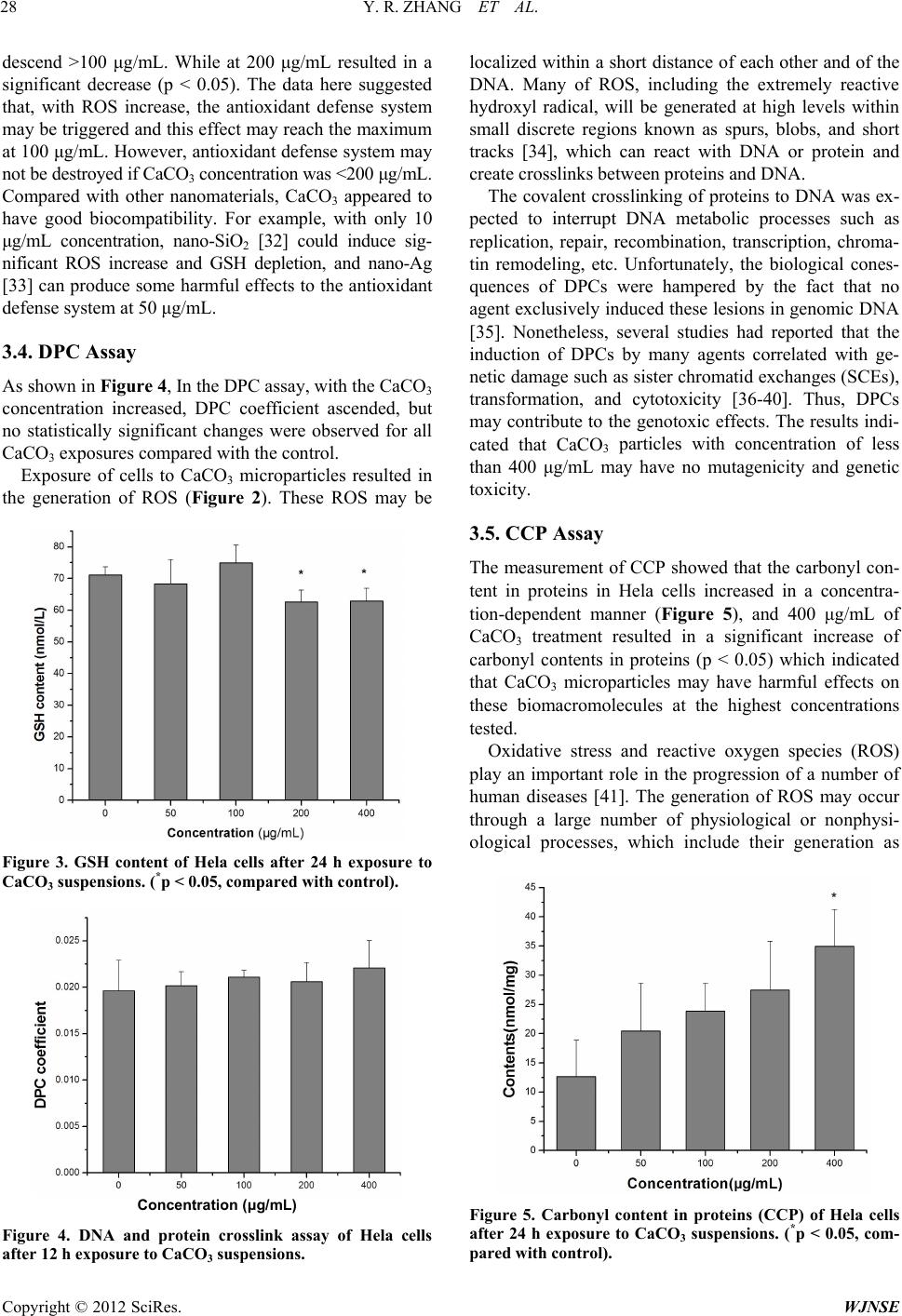 Y. R. ZHANG ET AL. 28 descend >100 μg/mL. While at 200 μg/mL resulted in a significant decrease (p < 0.05). The data here suggested that, with ROS increase, the antioxidant defense system may be triggered and this effect may reach the maximum at 100 μg/mL. However, antioxidant defense system may not be destroyed if CaCO3 concentration was <200 μg/mL. Compared with other nanomaterials, CaCO3 appeared to have good biocompatibility. For example, with only 10 μg/mL concentration, nano-SiO2 [32] could induce sig- nificant ROS increase and GSH depletion, and nano-Ag [33] can produce some harmful effects to the antioxidant defense system at 50 μg/mL. 3.4. DPC Assay As shown in Figure 4, In the DPC assay, with the CaCO3 concentration increased, DPC coefficient ascended, but no statistically significant changes were observed for all CaCO3 exposures compared with the control. Exposure of cells to CaCO3 microparticles resulted in the generation of ROS (Figure 2). These ROS may be Figure 3. GSH content of Hela cells after 24 h exposure to CaCO3 suspensions. (*p < 0.05, compared with control). Concentration (μg/mL) Figure 4. DNA and protein crosslink assay of Hela cells after 12 h exposure to CaCO3 suspensions. localized within a short distance of each other and of the DNA. Many of ROS, including the extremely reactive hydroxyl radical, will be generated at high levels within small discrete regions known as spurs, blobs, and short tracks [34], which can react with DNA or protein and create crosslinks between proteins and DNA. The covalent crosslinking of proteins to DNA was ex- pected to interrupt DNA metabolic processes such as replication, repair, recombination, transcription, chroma- tin remodeling, etc. Unfortunately, the biological cones- quences of DPCs were hampered by the fact that no agent exclusively induced these lesions in genomic DNA [35]. Nonetheless, several studies had reported that the induction of DPCs by many agents correlated with ge- netic damage such as sister chromatid exchanges (SCEs), transformation, and cytotoxicity [36-40]. Thus, DPCs may contribute to the genotoxic effects. The results indi- cated that CaCO3 particles with concentration of less than 400 μg/mL may have no mutagenicity and genetic toxicity. 3.5. CCP Assay The measurement of CCP showed that the carbonyl con- tent in proteins in Hela cells increased in a concentra- tion-dependent manner (Figure 5), and 400 μg/mL of CaCO3 treatment resulted in a significant increase of carbonyl contents in proteins (p < 0.05) which indicated that CaCO3 microparticles may have harmful effects on these biomacromolecules at the highest concentrations tested. Oxidative stress and reactive oxygen species (ROS) play an important role in the progression of a number of human diseases [41]. The generation of ROS may occur through a large number of physiological or nonphysi- ological processes, which include their generation as Figure 5. Carbonyl content in proteins (CCP) of Hela cells after 24 h exposure to CaCO3 suspensions. (*p < 0.05, com- pared with control). Copyright © 2012 SciRes. WJNSE
 Y. R. ZHANG ET AL. 29 by-products of normal cellular metabolism, primarily in the mitochondria. Excess ROS may damage all types of biological molecules. Oxidative damages to proteins, lip- ids, or DNA may all be seriously deleterious and may take place concomitantly [42]. 3.6. Cytotoxicity of CaCO3 Microparticles Drug delivery systems require biocompatible inorganic matrices that permit safe retention as well as controlled drug delivery. CaCO3 appear to fit these conditions. To evaluate the CaCO3 mcroparticles induced cyto- toxicity, an MTT assay was conducted to determine the viability of the treated Hela cells. As shown in Figure 6, even at the concentration of 400 μg/mL, the viabilities of the treated cells were 89.6%. Compared with MnO2 [30] at the same concentration the viabilities of Hela cells were only 32.45%. The results showed that porous sphe- rical CaCO3 microparticles had only a little cytotoxicity and could be applied as relatively safe drug vehicles. 4. Conclusion Results showed that at the high level of CaCO3 exposure, DCF-fluorescence intensity and carbonyl content in pro- teins(CCP) presented a significant increase compared with the control, DNA and protein crosslinks (DPC) coeffi- cient were also ascended, but no statistically significant changes were observed. Furthermore, GSH contents in Hela cells and cell viability were showed a significant decrease compared with the control. But compared with other nanomaterials, CaCO3 appeared to have good bio- compatibility. Therefore this paper implied that CaCO3 microparticles could be applied as relatively safe basic materials for drug vehicles, but with the caveat that the effects of high dosages should not be ignored when at- Figure 6. Results of MTT assay of Hela cells after 36 h ex- posure to CaCO3 suspensions. (*p < 0.05, compared with control). tempting to maximize therapeutic activity by increasing the concentration. 5. Acknowledgements This work was financially supported by a grant from the National Natural Science Foundation of China (Nos. 50802032, 20877202 and 20907075). REFERENCES [1] M. P. Ginebra, T. Traykova and J. A. Planell, “Calcium Phosphate Cements as Bone Drug Delivery Systems: A Review,” Journal of Controlled Release, Vol. 113, No. 2, 2006, pp. 102-110. doi:10.1016/j.jconrel.2006.04.007 [2] G. F. Paciotti, D. G. I. Kingston and L. Tamarkin, “Col- loidal Gold Nanoparticles: A Novel Nanoparticle Plat- form for Developing Multifunctional Tumor-Targeted Drug Delivery Vectors,” Nanobiotechnology, Drug Development Research, Vol. 67, No. 1, 2006, pp. 47-54. [3] A. Bianco, K. Kostarelos and M. Prato, “Applications of Carbon Nanotubes in Drug Delivery,” Current Opinion in Chemical Biology, Vol. 9, No. 6, 2005, pp. 674-679. doi:10.1016/j.cbpa.2005.10.005 [4] E. J. Anglin, L. Cheng, W. R. Freeman and M. J. Sailor, “Porous Silicon in Drug Delivery Devices and Materials,” Advanced Drug Delivery Reviews, Vol. 60, No. 11, 2008, pp. 1266-1277. doi:10.1016/j.addr.2008.03.017 [5] T. K. Jain, M. A. Morales, S. K. Sahoo, D. L. Leslie- Pelecky and V. Labhasetwar, “Iron Oxide Nanoparticles for Sustained Delivery of Anticancer Agents,” Molecular pharmaceutics, Vol. 2, No. 3, 2005, pp. 194-205. doi:10.1021/mp0500014 [6] Y. Li, D. Liu, H. Ai, Q. Chang, D. Liu, Y. Xia, S. Liu, N. Peng, Z. Xi and X. Yang, “Biological Evaluation of Lay- ered Double Hydroxides as Efficient Drug Vehicles,” Nanotechnology, Vol. 21, No. 10, 2010, p. 105101. [7] W. Wei, G. Ma, G. Hu, D. Yu, T. Mcleish, Z. Su and Z. Shen, “Preparation of Hierarchical Hollow CaCO3 Parti- cles and the Application as Anticancer Drug Carrier,” Journal of the American Chemical Society, Vol. 130, No. 47, 2008, pp. 15808-15810. doi:10.1021/ja8039585 [8] Q. Zhao, B. Han, Z. Wang and C. Gao, “Hollow Chitosan- Alginate Multilayer Microcapsules as Drug Delivery Ve- hicle: Doxorubicin Loading and in Vitro and in Vivo Studies,” Nanomedicine: Nanotechnology, Biology, and Medicine, Vol. 3, No. 1, 2007, pp. 63-74. doi:10.1016/j.nano.2006.11.007 [9] G. B. Sukhorukov, D. V. Volodkin and A. M. Günther, A. I. Petrov, D. B. Shenoy and H. Möhwald, “Porous Cal- cium Carbonate Microparticles as Templates for Encap- sulation of Bioactive Compounds,” Journal of Materials Chemistry, Vol. 14, 2004, pp. 2073-2081. doi:10.1039/b402617a [10] Y. Ueno, H. Futagawa, Y. Takagi, A. Ueno and Y. Mi- zushima, “Drug-Incorporating Calcium Carbonate Nano- particles for a New Delivery System,” Journal of Con- trolled Release, Vol. 103, No. 1, 2005, pp. 93-98. Copyright © 2012 SciRes. WJNSE
 Y. R. ZHANG ET AL. 30 doi:10.1016/j.jconrel.2004.11.015 [11] C. Wang, C. He, Z. Tong, X. Liu, B. Ren and F. Zeng, “Combination of Adsorption by Porous CaCO3 Micropar- ticles and Encapsulation by Polyelectrolyte Multilayer Films for Sustained Drug Delivery,” International Jour- nal of Pharmaceutics, Vol. 308, No. 1-2, 2006, pp. 160- 167. doi:10.1016/j.ijpharm.2005.11.004 [12] C. Peng, Q. Zhao and C. Gao, “Sustained Delivery of Doxorubicin by Porous CaCO3 and Chitosan/Alginate Multilayers-Coated CaCO3 Microparticles,” Colloids and Surfaces A: Physicochemical and Engineering, Vol. 353, No. 2-3, 2010, pp. 132-139. doi:10.1016/j.colsurfa.2009.11.004 [13] C. Buzea, I. I. Pacheco and K. Robbie, “Nanomaterials and Nanoparticles: Sources and Toxicity,” Biointerphases, Vol. 2, No. 4, 2007, pp. 17-71. doi:10.1116/1.2815690 [14] I. Linkov, F. Kyle and M. Lisa, “Nanotoxicology and Nanomedicine: Making Hard Decisions,” Nanomedcine, Vol. 2, No. 2, 2008, pp. 167-171. doi:10.1016/j.nano.2008.01.001 [15] H. Meng, Z. Chen, G. Xing, H. Yuan, C. Chen, F. Zhao, C. Zhang and Y. Zhao, “Ultrahigh Reactivity Provokes Nanotoxicity: Explanation of Oral Toxicity of Nano- Copper Particles,” Toxicology Letters, Vol. 175, No. 1-3, 2007, pp. 102-110. doi:10.1016/j.toxlet.2007.09.015 [16] W. Lin, Y. W. Huang, X. D. Zhou and Y. Ma, “In Vitro of Silica Nanoparticles in Human Lung Cancer Cells,” Toxicology and Applied Pharmacology, Vol. 217, No. 3, 2006, pp. 252-259. doi:10.1016/j.taap.2006.10.004 [17] K. Govindaraju, J. Shan, K. Levesque, S. N. A. Hussain, W. S. Powell and D. H. Eidelman, “Nitration of Respira- tory Epithelial Cells by Myeloperoxidase Depends on Extracellular Nitrite,” Nitric Oxide, Vol. 18, No. 3, 2008, pp. 184-194. doi:10.1016/j.niox.2008.01.004 [18] M. Audebert, B. Salles and P. Calsou, “Effect of Double- Strand Break DNA Sequence on the PARP-1 NHEJ Path- way,” Biochemical and Biophysical Research Communi- cations, Vol. 369, No. 3, 2008, pp. 982-988. doi:10.1016/j.bbrc.2007.11.132 [19] S. A. Mouron, C. A. Grillo, F. N. Dulout and C. D. Goli- jow, “Induction of DNA Strand Breaks, DNA-Protein Crosslinks and Sister Chromatid Exchanges by Arsenite in a Human Lung Cell Line,” Toxicology in Vitro, Vol. 20, No. 3, 2006, pp. 279-285. doi:10.1016/j.tiv.2005.07.005 [20] K. Pulskamp, S. Diabate and H. F. Krug, “Carbon Nano- tubes Show No Sign of Acute Toxicity but Induce Intra- cellular Reactive Oxygen Species in Dependence on Con- taminants,” Toxicology Letters, Vol. 168, No. 1, 2007, pp. 58-74. doi:10.1016/j.toxlet.2006.11.001 [21] Y. S. Liu, C. M. Li, Z. S. Lu and S. M. Ding, “Studies on Formation and Repair of Formaldehyde-Damaged DNA by Detection of DNA-Protein Crosslinks and DNA Breaks,” Frontiers in Bioscience, Vol. 11, 2006, pp. 991-997. doi:10.2741/1856 [22] A. R. Collins, “The Comet Assay for DNA Damage and Repair,” Molecular Biotechnology, Vol. 26, No. 3, 2004, pp. 249-261. doi:10.1385/MB:26:3:249 [23] S. Chen, L. S. Chang and Y. Wei, “Oxidative Damage to Proteins and Decrease of Antioxidant Capacity in Patients with Varicocele,” Free Radical Biology & Medicine, Vol. 30, No. 11, 2001, pp. 1328-1334. doi:10.1016/S0891-5849(01)00536-6 [24] G. Oberdorster, E. Oberdorster and J. Oberdorster, “Nano- toxicology: An Emerging Discipline Evolving from Stu- dies of Ultrafine Particles,” Environ Health Perspect, Vol. 113, 2005, pp. 823-839. doi:10.1289/ehp.7339 [25] D. K. Das, N. Maulik, M. Sato and P. S. Ray, “Reactive Oxygen Species Function as Second Messenger during Ischemic Preconditioning of Heart,” Molecular and Cel- lular Biochemistry, Vol. 196, No. 1-2, 1999, pp. 59-67. doi:10.1023/A:1006966128795 [26] V. E. Kagan, Y. Y. Tyurina, V. A. Tyurin, N. V. Konduru, A. I. Potapovich, A. N. Osipov, E. R. Kisin, D. Schwe- gler-Berry, R. Mercer, V. Castranova and A. A. Shvedova, “Direct and Indirect Effects of Single Walled Carbon Nanotubes on RAW 264.7 Macrophages: Role of Iron,” Toxicology Letters, Vol. 165, No. 1, 2006, pp. 88-100. doi:10.1016/j.toxlet.2006.02.001 [27] M. T. Zhu, W. Y. Feng, B. Wang, T. C. Wang, Y. Q. Gu, M. Wang, H. Q. Yang, Y. L. Zhao and Z. F. Chai, “Com- parative Study of Pulmonary Responses to Nano-and Submicron-Sized Ferric Oxide in Rats,” Toxicology, Vol. 247, No. 2-3, 2008, pp. 102-111. doi:10.1016/j.tox.2008.02.011 [28] T. C. Long, N. Saleh, R. D. Tilton, G. V. Lowry and B. Veronesi, “Titanium Dioxide (P25) Produces Reactive Oxygen Species in Immortalized Brain Microglia (BV2): Implications for Nanoparticle Neurotoxicity,” Environ- mental Science and Technology, Vol. 40, No. 14, 2006, pp. 4346-4352. doi:10.1021/es060589n [29] B. Wang, W. Y. Feng, M. Wang, T. C. Wang, Y. Q. Gu, M. T. Zhu, H. Q. Yang, J. W. Shi, F. Zhang, Y. L. Zhao, Z. F. Chai, H. F.Wang and J. Wang, “Acute Toxicological Impact of Nano- and Submicro-Scaled Zinc Oxide Pow- der on Healthy Adult Mice,” Journal of Nanoparticle Re- search, Vol. 10, No. 2, 2008, pp. 263-276. doi:10.1007/s11051-007-9245-3 [30] Y. Li, X. Tian, Z. Lu, C. Yang, G. Yang, X. Zhou, H. Yao, Z. Zhu, Z. Xi and X. Yang, “Mechanism for α- MnO2 Nanowire Induced Cytotoxicity in Hela Cells,” Journal of Nanoscience and Nanotechnology, Vol. 10, No. 1, 2010, pp. 1-8. doi:10.1166/jnn.2010.1719 [31] B. P. Yu, “Cellular Defenses against Damage from Reac- tive Oxygen Species,” Physiological Reviews, Vol. 74, No. 1, 1994, pp. 139-162. [32] W. S. Lin, Y. W. Huang, X. D. Zhou and Y. F. Ma, “In Vitro Toxicity of Silica Nanoparticles in Human Lung Cancer Cells,” Toxicology and Applied Pharmacology, Vol. 217, No. 3, 2006, pp. 252-259. doi:10.1016/j.taap.2006.10.004 [33] S. M. Hussain, K. L. Hess, J. M. Gearhart, K. T. Geiss and J. J. Schlager, “In Vitro Toxicity of Nanoparticles in BRL 3A Rat Liver Cells,” Toxicology in Vitro, Vol. 19, No. 7, 2005, pp. 975-983. doi:10.1016/j.tiv.2005.06.034 [34] A. Mozumder, “Early Production of Radicals from Charged Particle Tracks in Water,” Radiation Research, Vol. 104, 1985, pp. S33-S39. doi:10.2307/3576629 Copyright © 2012 SciRes. WJNSE
 Y. R. ZHANG ET AL. Copyright © 2012 SciRes. WJNSE 31 [35] S. Barker, M. Weinfeld and D. Murray, “DNA-Protein Crosslinks: Their Induction, Repair, and Biological Con- sequences,” Mutation Research, Vol. 589, No. 2, 2005, pp. 111-135. doi:10.1016/j.mrrev.2004.11.003 [36] M. O. Bradley, I. C. Hsu and C. C. Harris, “Relationship between Sister Chromatid Exchange and Mutagenicity, Toxicity and DNA Damage,” Nat ure, Vol. 282, 1979, pp. 318-320. doi:10.1038/282318a0 [37] M. O. Bradley and K. W. Kohn, “X-Ray Induced DNA Double Strand Break Production and Repair in Mammal- ian Cells as Measured by Neutral Filter Elution,” Nucleic Acids Research, Vol. 7, No. 3, 1979, pp. 793-804. doi:10.1093/nar/7.3.793 [38] A. J. Fornace and J. B. Little, “Malignant Transformation by the DNA-Protein Crosslinking Agent Trans-Pt (II) Diam- minedichloride,” Carcinogenesis, Vol. 1, No. 12, 1980, pp. 989-994. doi:10.1093/carcin/1.12.989 [39] A. J. Fornace, “Detection of DNA Single-Strand Breaks Produced during the Repair of Damage by DNA-Protein Crosslinking Agents,” Cancer Research, Vol. 42, 1982, pp. 145-149. [40] O. Merk and G. Speit, “Significance of Formaldehyde-In- duced DNA-Protein Crosslinks for Mutagenesis,” Envi- ronmental and Molecular Mutagenesis, Vol. 32, No. 3, 1998, pp. 260-268. doi:10.1002/(SICI)1098-2280(1998)32:3<260::AID-EM9 >3.0.CO;2-M [41] B. Halliwell and J. Gutteridge, “Free Radicals in Biology and Medicine,” Oxford University, Oxford, 1999. [42] I. D. Donnea, R. Rossib, D. Giustarinib, A. Milzania and R. Colomboa, “Protein Carbonyl Groups as Biomarkers of Oxidative Stress,” Clinica Chimica Acta, Vol. 329, No. 1-2, 2003, pp. 23-38. doi:10.1016/S0009-8981(03)00003-2
|