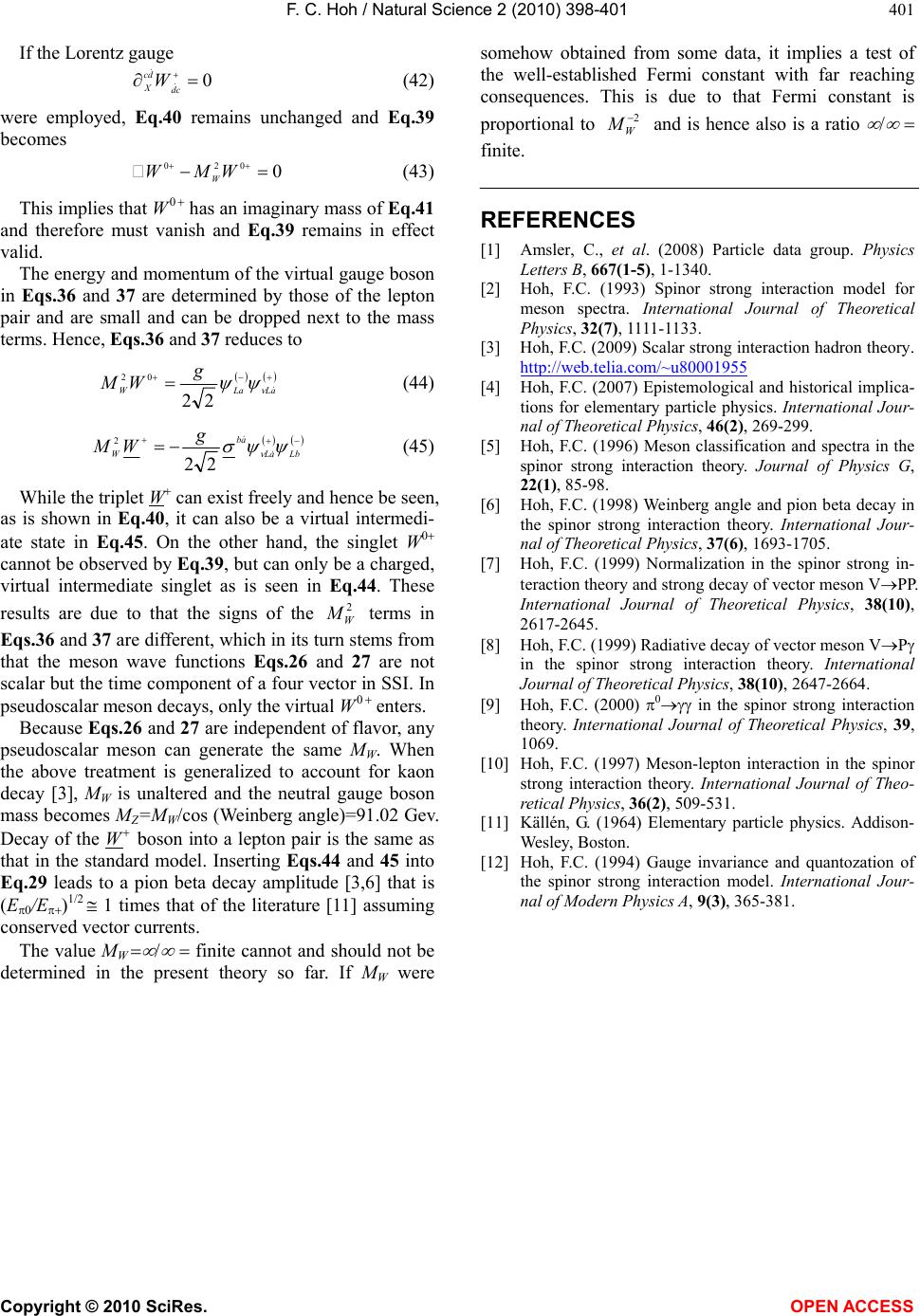
F. C. Hoh / Natural Science 2 (2010) 398-401
Copyright © 2010 SciRes. OPEN ACCESS
401
If the Lorentz gauge
0
cd
dc
XW
(42)
were employed, Eq.40 remains unchanged and Eq.39
becomes
0
020 WMW W (43)
This implies that W
has an imaginary mass of Eq.41
and therefore must vanish and Eq.39 remains in effect
valid.
The energy and momentum of the virtual gauge boson
in Eqs.36 and 37 are determined by those of the lepton
pair and are small and can be dropped next to the mass
terms. Hence, Eqs.36 and 37 reduces to
aLLaW
g
WM
22
02 (44)
LbaL
ab
W
g
WM
22
2 (45)
While the triplet W can exist freely and hence be seen,
as is shown in Eq.40, it can also be a virtual intermedi-
ate state in Eq.45 . On the other hand, the singlet W
cannot be observed by Eq.39, but can only be a charged,
virtual intermediate singlet as is seen in Eq.44 . These
results are due to that the signs of the 2
W
terms in
Eqs.36 and 37 are different, which in its turn stems from
that the meson wave functions Eqs.26 and 27 are not
scalar but the time component of a four vector in SSI. In
pseudoscalar meson decays, only the virtual W
enters.
Because Eqs.26 and 27 are independent of flavor, any
pseudoscalar meson can generate the same MW. When
the above treatment is generalized to account for kaon
decay [3], MW is unaltered and the neutral gauge boson
mass becomes MZ=MW/cos (Weinberg angle)=91.02 Gev.
Decay of the W boson into a lepton pair is the same as
that in the standard model. Inserting Eqs.44 and 45 into
Eq.29 leads to a pion beta decay amplitude [3,6] that is
(E0/E)1/2 1 times that of the literature [11] assuming
conserved vector currents.
The value MW
finite cannot and should not be
determined in the present theory so far. If MW were
somehow obtained from some data, it implies a test of
the well-established Fermi constant with far reaching
consequences. This is due to that Fermi constant is
proportional to 2
W
and is hence also is a ratio
finite.
REFERENCES
[1] Amsler, C., et al. (2008) Particle data group. Physics
Letters B, 667(1-5), 1-1340.
[2] Hoh, F.C. (1993) Spinor strong interaction model for
meson spectra. International Journal of Theoretical
Physics, 32(7), 1111-1133.
[3] Hoh, F.C. (2009) Scalar strong interaction hadron theory.
http://web.telia.com/~u80001955
[4] Hoh, F.C. (2007) Epistemological and historical implica-
tions for elementary particle physics. International Jour-
nal of Theoretical Physics, 46(2), 269-299.
[5] Hoh, F.C. (1996) Meson classification and spectra in the
spinor strong interaction theory. Journal of Physics G,
22(1), 85-98.
[6] Hoh, F.C. (1998) Weinberg angle and pion beta decay in
the spinor strong interaction theory. International Jour-
nal of Theoretical Physics, 37(6), 1693-1705.
[7] Hoh, F.C. (1999) Normalization in the spinor strong in-
teraction theory and strong decay of vector meson VPP.
International Journal of Theoretical Physics, 38(10),
2617-2645.
[8] Hoh, F.C. (1999) Radiative decay of vector meson VP
in the spinor strong interaction theory. International
Journal of Theoretical Physics, 38(10), 2647-2664.
[9] Hoh, F.C. (2000) 0 in the spinor strong interaction
theory. International Journal of Theoretical Physics, 39,
1069.
[10] Hoh, F.C. (1997) Meson-lepton interaction in the spinor
strong interaction theory. International Journal of Theo-
retical Physics, 36(2), 509-531.
[11] Källén, G. (1964) Elementary particle physics. Addison-
Wesley, Boston.
[12] Hoh, F.C. (1994) Gauge invariance and quantozation of
the spinor strong interaction model. International Jour-
nal of Modern Physics A, 9(3), 365-381.