 Vol.3, No.1, 16-28 (2012) Journ al of Biophysical Chemistry http://dx.doi.org/10.4236/jbpc.2012.31002 Steady-state kinetics of Roystonea regia palm tree peroxidase Laura Sánchez Zamorano1, Nazaret Hidalgo Cuadrado1, Patricia Pérez Galende1, Manuel G. Roig1*, Valery L. Shnyrov2 1Departamento de Química Física, Facultad de Química, Universidad de Salamanca, Salamanca, Spain; *Corresponding Author: mgr@usal.es 2Departamento de Bioquímica y Biología Molecular, Facultad de Biología, Universidad de Salamanca, Salamanca, Spain Received 12 October 2011; revised 20 November 2011; accepted 5 December 2011 ABSTRACT Royal palm tree peroxidase (RPTP) has been isolated to homogeneity from leaves of Roys- tonea regia palm trees. The enz yme puri fication steps included homogenization, (NH4)SO4 pre- cipitation, extraction of palm leaf colored com- pounds and consecutive chromatography on Ph- enyl-Sepharose, TSK-Gel DEAE-5PW and Super- dex-200. The novel perox idase w as characterized as having a molecular weight of 48.2 ± 3.0 kDa and an isoelectric point pI 5.4 ± 0.1. The enzyme forms dimers in solution with approximate mo- lecular weight of 92 ± 2 kDa. Here we invest igated the steady-state kinetic mechanism of the H2O2- supported oxidation of different organic substr- ates by RPTP. The results of the analysis of the initial rates vs. H2O2 and reducing substrate con- centrations were seen to be consistent with a substrate-inhibited Ping-Pong Bi-Bi reaction me- chanism. The phenomenological approach used expresses the peroxidase Ping-Pong mechanism in the form of the Michaelis-Menten equation and affords an interpretation of the effects in terms of the kinetic parameters K, , kcat, , and of the microscopic rate constants k1 and k3 of the shared three-step peroxidase cata- lytic cycle. Furthermore, the concentration and time-dependences and the mechanism of the sui- cide inactivation of RPTP by hydrogen peroxide were studied kinetically with guaiacol as co-sub- strate. The turnover number (r) of H2O2 re quired to complete the inactivation of the enzyme was 2154 ± 100 and t he apparent rate constants of catalysis 185 s–1 and 18 s–1. 22 HO mK2 AH mK22 HO SI K2 AH SI Keywords: Roystonea regia; Peroxidase; Steady-State Kinetics; Substrate Inhibition; Mechanism-Based Inactivatio n Ki ne ti cs ; Hydrogen Peroxide 1. INTRODUCTION Peroxidases (EC 1.11.1.7; donor: hydrogen peroxide oxidoreductase) are widely distributed in the living world and are involved in many physiological processes. The oxidation of many biological substances in body fluids leads to the production of a certain amount of hydrogen peroxide. Thus, although the function of peroxidases is often seen mainly in terms of causing the conversion of toxic H2O2 to H2O, their wider participation in other re- actions, such as cell wall formation, lignification, the protection of tissues from pathogenic microorganisms, suberization, auxin catabolism, defense, stress, etc., should not be overlooked [1]. Apart from their biological functions, peroxidases are important as regards many biotechnological applications. This group of enzymes, especially those of plant origin, enjoys widespread use as catalysts for phenolic resin synthesis [2,3] as indicators for food processing and di- agnostic reagents [4,5], and as additives in bioremedia- tion [6,7]. Under certain specific conditions, the radicals formed can break bonds in polymeric materials, destroy- ing them [8]. Peroxidases reduce hydrogen peroxide and oxidize a large number of compounds, including phenols, aromatic amines, thiosanisoles, halide and thyocianate ions, and fatty acids. Their selectivity with respect to reducing substrates depends on their specific type [9]. Since they are able to oxidize a broad variety of organic and inor- ganic substrates [8], it is somewhat difficult to determine which substrates are physiologically relevant for plant peroxidases. Peroxidases have been identified throughout the plant kingdom, but that from horseradish root (HRP), and in particular the slightly basic C isoenzyme (HRPC), is the one that has attracted the most attention. Despite this, recently more data have become available regarding Copyright © 2012 SciRes. OPEN ACCESS
 L. S. Zamorano et al. / Journal of Biophysical Chemistry 3 (2012) 16-28 17 peroxidases from other plants, such as peanut [10], bar- ley [11], tea [12], Arabidopsis thaliana [13], and palm trees [14-18]. The shared three-step catalytic cycle of peroxidases, involving different intermediate enzyme forms, is known as the Poulos-Kraut mechanism [19,20]. Catalysis is ini- tiated by the binding of H2O2 to the high-spin ferric haem iron of resting peroxidase, followed by heterolytic cleav- age of the peroxide oxygen-oxygen bond under the in- fluence of highly conserved histidine and arginine resi- dues at the active site [21]. The haem undergoes a two- electron oxidation, forming an intermediate (Compound I) that contains an oxyferryl species (Fe(IV)=O) and a por- phyrin π-cation radical. As the co-product of the reac- tion, a water molecule is generated. Completion of the catalytic cycle usually involves two successive single- electron transfers from separate reducing substrate mole- cules to the enzyme. The first reduction, of the porphyrin π-cation radical in Compound I, yields a second enzyme intermediate, Compound II, which retains the iron in the oxyferryl state [22]. Under steady-state conditions, the reduction of Compound II, to recover the ferric enzyme, is often rate-limiting. The extremely reactive free radi- cals released from the catalytic cycle often condense spontaneously, giving rise to polymers. The peroxidase cycle is generally considered irre- versible. Nevertheless, it is unquestionable that adsorp- tion complexes between the enzyme and its substrates exist physically [23]. The microscopic constants govern- ing the equilibrium between aromatic compounds and peroxidase have been estimated. Even though the co- substrates (donor or H2O2) in the enzyme modulate each other’s affinity, it is possible for the mechanism to pro- ceed via random binding. This observation, together with retain special kinetic features [24], supports the notion that there is no need for the peroxide to bind to the en- zyme prior to donor adsorption. Here we investigated the kinetic mechanism of the H2O2-supported oxidation of different organic substrates by means of a novel plant peroxidase from the Roystonea regia palm tree (RPTP). Among the broad variety of organic and inorganic substrates of peroxidases, in the present work we ex- plored six organic chromogenic substrates: three pheno- lics, guaiacol (2-metoxiphenol), catechol (2-hydroxyphe- nol) and ferulic acid (3-(4-hydroxy-3-methoxyphenyl)-2- propenoic acid), and ABTS (2,2’-azino-bis(3-ethylbenz- thiazoline-6-sulphonic acid), often used as a reference substrate, o-dianisidine and o-phenylendiamine, the latter three suitable for use in ELISA procedures that employ peroxidase conjugates [25-28]. Since these oxidation reactions exhibit Michaelis- Menten saturation kinetics with respect to both substrates, the chromogenic substrate and H2O2, the system was amenable to steady-state kinetic experiments, which were used to deduce the kinetic mechanism of the reac- tion following methodologies established for two-sub- strate enzyme systems [29]. The results of the initial-rate and inhibition studies carried out here indicate that the H2O2-supported oxidation of different organic substrates catalyzed by this peroxidase proceeds via a Ping-Pong Bi-Bi mechanism mediated by the oxidized enzyme in- termediate Compounds I and II. Palm peroxidase catalyzes the oxidation reactions of a large variety of reducing substrates, using H2O2 as oxi- dizing agent [19,30,31]. In the absence of reducing sub- strates, excess H2O2 leads to the inactivation of the en- zyme, in this case acting as a suicide substrate of per- oxidase and being irreversibly bound to its active site [32, 33]. Despite this, it has been suggested that HRP inacti- vation by hydrogen peroxide would be due to the forma- tion of one or several non-active enzyme products, pro- bably through the formation of Compound III (peroxyl- FeIII porphyrin) [34,35]. The oxidative inactivation of peroxidases is mecha- nism-based. The molecular mechanism driving this hy- drogen peroxide-mediated inactivation is extraordinarily complex because an array of reactions can occur subse- quent to the reaction of the haem iron with the hydrop- eroxide. Regardless of the differences among the per- oxidases, a common inactivation mechanism involving several stages can be proposed. In the absence of sub- strate, or when they are exposed to high hydrogen per- oxide concentrations, peroxidases show the kinetic be- havior of suicide inactivation, in which hydrogen perox- ide is the suicide substrate that converts Compound II into a highly reactive peroxy-iron(III) porphyrin free- radical termed Compound III [36]. Compound III does not form part of the peroxidase cycle, but is produced under excessive exposure of protonated Compound II to oxidative species in a reaction that is partially mediated by free superoxide radicals [37]. Despite representing different structural groups, ki- netic models for the hydrogen peroxide-mediated inacti- vation of horseradish peroxidase (HRP) [33], ascorbate peroxidase (APX) [38], peroxidase from the Royal Palm Tree (RPTP) [16], microperoxidase-11 [39] and Chamae- rops excelsa peroxidase (CEP) [40] are similar in the sense that they are time-dependent and exhibit saturation kinetics. From the inactivation stoichiometry, it has been concluded that for APX only 2.5 molecules of hydrogen peroxide are required per active site for the inactivation form to be generated [38], in contrast to the 265 mole- cules required for HRP [33]. This difference is due to the low catalytic activity of HRP, which is absent in APX [41]. For APX peroxidase, inactivation is correlated with enzyme bleaching, suggesting haem destruction [38]. Another factor in this difference is the glycosylation of Copyright © 2012 SciRes. OPEN ACCES S
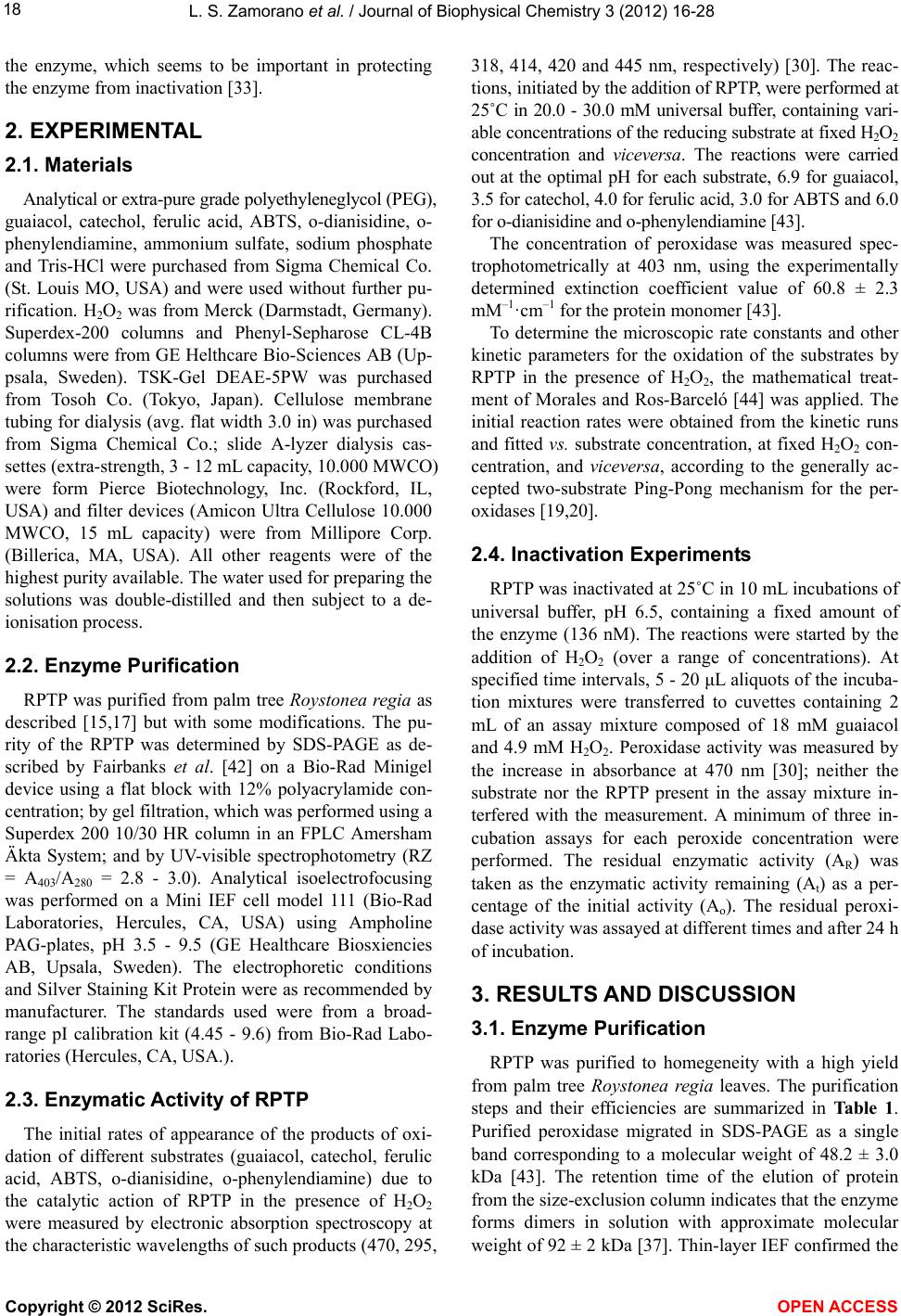 L. S. Zamorano et al. / Journal of Biophysical Chemistry 3 (2012) 16-28 18 the enzyme, which seems to be important in protecting the enzyme from inactivation [33]. 2. EXPERIMENTAL 2.1. Materials Analytical or extra-pure grade polyethyleneglycol (PEG), guaiacol, catechol, ferulic acid, ABTS, o-dianisidine, o- phenylendiamine, ammonium sulfate, sodium phosphate and Tris-HCl were purchased from Sigma Chemical Co. (St. Louis MO, USA) and were used without further pu- rification. H2O2 was from Merck (Darmstadt, Germany). Superdex-200 columns and Phenyl-Sepharose CL-4B columns were from GE Helthcare Bio-Sciences AB (Up- psala, Sweden). TSK-Gel DEAE-5PW was purchased from Tosoh Co. (Tokyo, Japan). Cellulose membrane tubing for dialysis (avg. flat width 3.0 in) was purchased from Sigma Chemical Co.; slide A-lyzer dialysis cas- settes (extra-strength, 3 - 12 mL capacity, 10.000 MWCO) were form Pierce Biotechnology, Inc. (Rockford, IL, USA) and filter devices (Amicon Ultra Cellulose 10.000 MWCO, 15 mL capacity) were from Millipore Corp. (Billerica, MA, USA). All other reagents were of the highest purity available. The water used for preparing the solutions was double-distilled and then subject to a de- ionisation process. 2.2. Enzyme Purification RPTP was purified from palm tree Roystonea regia as described [15,17] but with some modifications. The pu- rity of the RPTP was determined by SDS-PAGE as de- scribed by Fairbanks et al. [42] on a Bio-Rad Minigel device using a flat block with 12% polyacrylamide con- centration; by gel filtration, which was performed using a Superdex 200 10/30 HR column in an FPLC Amersham Äkta System; and by UV-visible spectrophotometry (RZ = A403/A280 = 2.8 - 3.0). Analytical isoelectrofocusing was performed on a Mini IEF cell model 111 (Bio-Rad Laboratories, Hercules, CA, USA) using Ampholine PAG-plates, pH 3.5 - 9.5 (GE Healthcare Biosxiencies AB, Upsala, Sweden). The electrophoretic conditions and Silver Staining Kit Protein were as recommended by manufacturer. The standards used were from a broad- range pI calibration kit (4.45 - 9.6) from Bio-Rad Labo- ratories (Hercules, CA, USA.). 2.3. Enzymatic Activity of RPTP The initial rates of appearance of the products of oxi- dation of different substrates (guaiacol, catechol, ferulic acid, ABTS, o-dianisidine, o-phenylendiamine) due to the catalytic action of RPTP in the presence of H2O2 were measured by electronic absorption spectroscopy at the characteristic wavelengths of such products (470, 295, 318, 414, 420 and 445 nm, respectively) [30]. The reac- tions, initiated by the addition of RPTP, were performed at 25˚C in 20.0 - 30.0 mM universal buffer, containing vari- able concentrations of the reducing substrate at fixed H2O2 concentration and viceversa. The reactions were carried out at the optimal pH for each substrate, 6.9 for guaiacol, 3.5 for catechol, 4.0 for ferulic acid, 3.0 for ABTS and 6.0 for o-dianisidine and o-phenylendiamine [43]. The concentration of peroxidase was measured spec- trophotometrically at 403 nm, using the experimentally determined extinction coefficient value of 60.8 ± 2.3 mM–1·cm–1 for the protein monomer [43]. To determine the microscopic rate constants and other kinetic parameters for the oxidation of the substrates by RPTP in the presence of H2O2, the mathematical treat- ment of Morales and Ros-Barceló [44] was applied. The initial reaction rates were obtained from the kinetic runs and fitted vs. substrate concentration, at fixed H2O2 con- centration, and viceversa, according to the generally ac- cepted two-substrate Ping-Pong mechanism for the per- oxidases [19,20]. 2.4. Inactivation Experiments RPTP was inactivated at 25˚C in 10 mL incubations of universal buffer, pH 6.5, containing a fixed amount of the enzyme (136 nM). The reactions were started by the addition of H2O2 (over a range of concentrations). At specified time intervals, 5 - 20 μL aliquots of the incuba- tion mixtures were transferred to cuvettes containing 2 mL of an assay mixture composed of 18 mM guaiacol and 4.9 mM H2O2. Peroxidase activity was measured by the increase in absorbance at 470 nm [30]; neither the substrate nor the RPTP present in the assay mixture in- terfered with the measurement. A minimum of three in- cubation assays for each peroxide concentration were performed. The residual enzymatic activity (AR) was taken as the enzymatic activity remaining (At) as a per- centage of the initial activity (Ao). The residual peroxi- dase activity was assayed at different times and after 24 h of incubation. 3. RESULTS AND DISCUSSION 3.1. Enzyme Purification RPTP was purified to homegeneity with a high yield from palm tree Roystonea regia leaves. The purification steps and their efficiencies are summarized in Table 1. Purified peroxidase migrated in SDS-PAGE as a single band corresponding to a molecular weight of 48.2 ± 3.0 kDa [43]. The retention time of the elution of protein from the size-exclusion column indicates that the enzyme forms dimers in solution with approximate molecular eight of 92 ± 2 kDa [37]. Thin-layer IEF confirmed the w Copyright © 2012 SciRes. OPEN ACCESS
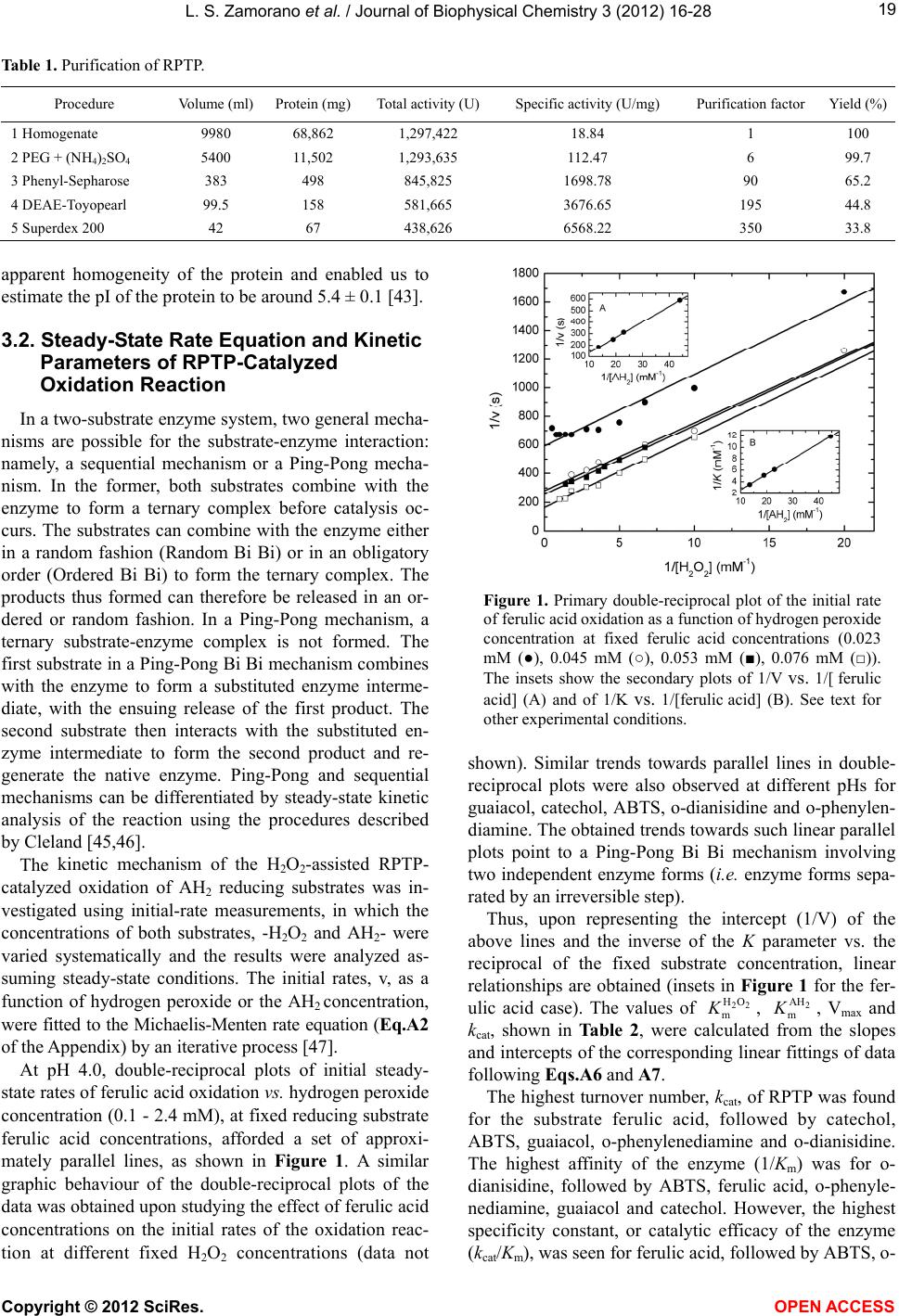 L. S. Zamorano et al. / Journal of Biophysical Chemistry 3 (2012) 16-28 Copyright © 2012 SciRes. 19 Table 1. Purification of RPTP. Procedure Volume (ml) Protein (mg) Total activity (U) Specific activity (U/mg) Purification factor Yield (%) 1 Homogenate 9980 68,862 1,297,422 18.84 1 100 2 PEG + (NH4)2SO4 5400 11,502 1,293,635 112.47 6 99.7 3 Phenyl-Sepharose 383 498 845,825 1698.78 90 65.2 4 DEAE-Toyopearl 99.5 158 581,665 3676.65 195 44.8 5 Superdex 200 42 67 438,626 6568.22 350 33.8 apparent homogeneity of the protein and enabled us to estimate the pI of the protein to be around 5.4 ± 0.1 [43]. 3.2. Steady-State Rate Equation and Kinetic Parameters of RPTP-Catalyzed Oxidation Reaction In a two-substrate enzyme system, two general mecha- nisms are possible for the substrate-enzyme interaction: namely, a sequential mechanism or a Ping-Pong mecha- nism. In the former, both substrates combine with the enzyme to form a ternary complex before catalysis oc- curs. The substrates can combine with the enzyme either in a random fashion (Random Bi Bi) or in an obligatory order (Ordered Bi Bi) to form the ternary complex. The products thus formed can therefore be released in an or- dered or random fashion. In a Ping-Pong mechanism, a ternary substrate-enzyme complex is not formed. The first substrate in a Ping-Pong Bi Bi mechanism combines with the enzyme to form a substituted enzyme interme- diate, with the ensuing release of the first product. The second substrate then interacts with the substituted en- zyme intermediate to form the second product and re- generate the native enzyme. Ping-Pong and sequential mechanisms can be differentiated by steady-state kinetic analysis of the reaction using the procedures described by Cleland [45,46]. Figure 1. Primary double-reciprocal plot of the initial rate of ferulic acid oxidation as a function of hydrogen peroxide concentration at fixed ferulic acid concentrations (0.023 mM (●), 0.045 mM (○), 0.053 mM (■), 0.076 mM (□)). The insets show the secondary plots of 1/V vs. 1/[ ferulic acid] (A) and of 1/K vs. 1/[ferulic acid] (B). See text for other experimental conditions. shown). Similar trends towards parallel lines in double- reciprocal plots were also observed at different pHs for guaiacol, catechol, ABTS, o-dianisidine and o-phenylen- diamine. The obtained trends towards such linear parallel plots point to a Ping-Pong Bi Bi mechanism involving two independent enzyme forms (i.e. enzyme forms sepa- rated by an irreversible step). The kinetic mechanism of the H2O2-assisted RPTP- catalyzed oxidation of AH2 reducing substrates was in- vestigated using initial-rate measurements, in which the concentrations of both substrates, -H2O2 and AH2- were varied systematically and the results were analyzed as- suming steady-state conditions. The initial rates, v, as a function of hydrogen peroxide or the AH2 concentration, were fitted to the Michaelis-Menten rate equation (Eq.A2 of the Appendix) by an iterative process [47]. Thus, upon representing the intercept (1/V) of the above lines and the inverse of the K parameter vs. the reciprocal of the fixed substrate concentration, linear relationships are obtained (insets in Figure 1 for the fer- ulic acid case). The values of , , Vmax and kcat, shown in Table 2, were calculated from the slopes and intercepts of the corresponding linear fittings of data following Eqs.A6 and A7. 22 HO m K2 AH m K At pH 4.0, double-reciprocal plots of initial steady- state rates of ferulic acid oxidation vs. hydrogen peroxide concentration (0.1 - 2.4 mM), at fixed reducing substrate ferulic acid concentrations, afforded a set of approxi- mately parallel lines, as shown in Figure 1. A similar graphic behaviour of the double-reciprocal plots of the data was obtained upon studying the effect of ferulic acid concentrations on the initial rates of the oxidation reac- tion at different fixed H2O2 concentrations (data not The highest turnover number, kcat, of RPTP was found for the substrate ferulic acid, followed by catechol, ABTS, guaiacol, o-phenylenediamine and o-dianisidine. The highest affinity of the enzyme (1/Km) was for o- dianisidine, followed by ABTS, ferulic acid, o-phenyle- nediamine, guaiacol and catechol. However, the highest specificity constant, or catalytic efficacy of the enzyme (kcat/Km), was seen for ferulic acid, followed by ABTS, o- OPEN A CCESS
 L. S. Zamorano et al. / Journal of Biophysical Chemistry 3 (2012) 16-28 20 Table 2. Kinetic parameters obtained for the H2O2-mediated oxidation of substrates by RPTP. See text for experimental conditions. Substrate Km (H2O2) (M) Km (AH2) (M) Vmax (M·s–1) [Eo] (10–10 M) kcat (s–1) kcat/Km (H2O2) (M–1·s–1) kcat/Km (AH2) (M–1·s–1) k1 (µM–1·s–1) k3 (µM–1·s–1) Guaiacol 2.7 × 10–3 15.2 × 10–3 1.2 × 10–6 5.61 2.1 × 1037.8 × 105 1.4 × 105 0.33 0.08 ABTS 1.8 × 10–3 4.9 × 10–4 2.0 × 10–6 6.05 3.3 × 1031.8 × 106 6.7 × 105 1.30 4.80 Ferulic acid 1.9 × 10–3 5.4 × 10–4 4.8 × 10–6 8.91 5.4 × 1042.8 × 107 1.0 × 108 1.82 6.96 o-Dianisidine 7.0 × 10-4 1.0 × 10–4 1.2 × 10–7 2.97 4.0 × 1025.7 × 105 4.0 × 106 0.25 1.91 o-Phenilendiamine 2.2 × 10–3 1.4 × 10–3 7.8 × 10–7 10.2 7.6 × 1023.5 × 105 5.5 × 105 0.23 0.26 Catechol 3.5 × 10–3 8.8 × 10–2 2.8 × 10–5 35.0 8.0 × 1032.3 × 106 9.0 × 104 0.40 0.02 dianisidine, o-phenylenediamine, guaiacol and catechol. Similar reactivities for these substrates have been found for African [30] and Chamaerops excelsa [48] palm tree peroxidases. 3.3. Microscopic Rate Constants Peroxidases catalyze the oxidation of AH2 organic substrates, using H2O2 (or other peroxides) as an electron acceptor in a three-step catalytic cycle involving differ- ent intermediate enzyme forms [19,20]: 1 22 2 EHOEIHO k (1) 2 2 EI AHEII AH k (2) 3 22 EIIAHEHO AH k (3) where E is the native enzyme. The monoelectronic oxi- dation of the native state E affords an intermediate state termed EI (Eq.1). EI is responsible for the oxidation of the electron-donor substrate (AH2), accepting one proton and one electron and generating its free radical (AH ), together with another enzyme state, designated EII (Eq.2). Finally, EII is reduced by a second molecule of sub- strate (Eq.3), giving rise to a second free radical (AH•). The microscopic constant k1 (the constant of EI forma- tion) indicates the reactivity of the enzyme towards hy- drogen peroxide, and k3 (the constant of EII reduction) represents the reactivity of the enzyme towards the re- ducing substrate. With a view to calculating the microscopic constants (k1 and k3) of the oxidation of the substrates by RPTP, the oxidation rates of the substrates were fitted for each concentration of AH2 and H2O2, assuming the steady- state approach and considering that k2 > k3. As may be seen in the Appendix, double-reciprocal plots (1/v vs. 1/[H2O2]) allowed us to calculate the A and B values for each AH2 concentration. Figure 2 shows the plot of A vs. B (Eq.A8) for four ferulic acid concentra- tions. From this straight line it is possible to calculate the value of k1 (formation constant of Compound I) for per- oxidase-mediated ferulic acid oxidation. The value ob- tained, as well as those obtained for guaiacol, catechol, ABTS, o-dianisidine and o-phenylendiamine, is shown in Table 2. As shown in the Appendix, double reciprocal plots (1/v vs. 1/[AH2]) allowed us to calculate the A and B values (Eq.A9) for each H2O2 concentration. Figure 3 shows the plot of A vs. B values for three H2O2 concen- trations during the oxidation of ferulic acid. With this plot it is possible to calculate the value of k3 (the forma- values (Eq.A9) for each H2O2 concentration. Figure 3 Figure 2. Secondary plot of parameters A (nmol·s–1) vs. B (mM) obtained by varying H2O2 concentra- tions for four ferulic acid concentrations (0.023, 0.045, 0.053, 0.076 mM). See text for other expe- rimental conditions. Figure 3. Secondary plot of parameters A (nmol·s–1) vs. B (mM) obtained by varying ferulic acid con- centrations for five H2O2 concentrations (0.1, 0.15, 0.30, 0.50, 1.00 mM). See text for other experi- mental conditions. Copyright © 2012 SciRes. OPEN ACCESS
 L. S. Zamorano et al. / Journal of Biophysical Chemistry 3 (2012) 16-28 21 shows the plot of A vs. B values for three H2O2 concen- trations during the oxidation of ferulic acid. With this plot it is possible to calculate the value of k3 (the forma- tion constant of Compound II) for peroxidase-mediated ferulic acid oxidation. The value obtained, as well as those obtained for guaiacol, catechol, ABTS, o-dianis- idine and o-phenylendiamine, is also listed in Table 2. These A vs. B plots allowed us to calculate the real re- action constants (ki) from steady-state measurements of the oxidation rate, avoiding their dependence on the sub- strate concentration [20]. From the rate constant values (ki) shown in Table 2, it may be deduced that the RPTP is capable of oxidizing phenolic and aromatic amine substrates. The data ob- tained show that the most reactive substrate for RPTP was ferulic acid, followed by ABTS, the aromatic amines o-dianisidine and o-phenylendiamine, guaiacol and cate- chol being the least reactive substrates. The high reactiv- ity of ABTS must be due to its greater number of H-bond acceptor atoms, because the highest values of reactivity constants are seen for substrates with the most acceptor H-bonds (www.chemicalregister.com). ABTS has ten H- bond acceptor sites; ferulic acid and o-dianisidine four, and o-phenylendiamine, guaiacol and catechol two. In respect of H-bond donor sites—ABTS, ferulic acid, o- dianisidine, o-phenylendiamine, catechol—each has two H-bond donor sites and guaiacol only one. Similar studies addressing kinetic parameters and mi- croscopic rate constants carried out with African [30] and Chamaerops excelsa [48] palm tree peroxidases have shown that these enzymes exhibit greater reactivity to- wards ferulic acid and ABTS, followed by the aromatic amines o-dianisidine, o-phenylendiamine and, finally, by phenolic substrates with one or two hydroxyl groups in their chemical structures. In contrast, both soybean and peanut peroxidases are more reactive towards guaiacol than towards amines [30,49]. Horseradish and tobacco peroxidases have been reported to be equally reactive towards guaiacol and o-dianisidine and about 10 - 15 times less reactive towards o-phenylendiamine [43]. Commonly substrate specificity studies of peroxidases are conducted with only one substrate present, apart from H2O2, in the reaction mixture at a given time; i.e. without any alternative substrates able to undergo the same reac- tion. This is because the presence of competing sub- strates tends to complicate the analysis, without provid- ing much more information than would be obtained by studying the substrates separately. However, this implies an important difference between experimental practice and the physiological conditions under which enzymes usually exist. Thus, most enzymes are not perfectly spe- cific for a single substrate and must often select between several that are available simultaneously. Therefore, to be physiologically meaningful enzyme specificity must be defined in terms of how well the enzyme can dis- criminate between the substrates present in the same re- action mixture. This does not mean that it cannot be de- termined from the kinetic parameters of the enzyme for separate substrates, but it does mean that these parame- ters need to be interpreted correctly and not on a casual basis [50]. 3.4. Substrate Inhibition In order to further check the kinetic mechanism of the substrate oxidation reactions catalyzed by RPTP, inhibi- tion studies were carried out. One of the characteristic features of Ping-Pong reaction mechanisms is the occur- rence of competitive substrate inhibition by both sub- strates [29]. In the Ping-Pong reaction mechanism, since the three forms of the enzyme—E, CoI and CoII—are so similar, it is reasonable to expect AH2 to have some affinity for E as well as CoI and CoII and, if the active sites in CoI and CoII are not too full for the adsorption of H2O2, for H2O2 to show some affinity for CoI and CoII [51]. In peroxi- dases, reports have been made of the formation of a non-productive, or dead-end, complex between AH2 and E and the reaction of high concentrations of H2O2 with CoI, affording H2O2 and O2 [52]. As stated in the Appendix, following the reciprocal of Eq.A13, plots of 1/v vs. 1/[H2O2] at fixed [AH2] should be linear and should intersect on the y axis. This graphi- cal behaviour was observed at fixed ferulic acid inhibit- tory concentrations (Figure 4). In this sense, Figure 4 (inset) shows this linear plot for ferulic acid. For the other substrates studied, a similar KN (mM·s) Figure 4. Primary double-reciprocal plot of the initial rate of ferulic acid oxidation as a function of hydrogen peroxide concentration at fixed ferulic acid inhibitory concentrations (0.095 mM (■), 0.104 mM (□), 0.113 mM (○), 0.128 mM (●)). The inset shows the secondary plot of K/V vs. ferulic acid concentration. See text for other experimental condi- tions. Copyright © 2012 SciRes. OPEN ACCES S
 L. S. Zamorano et al. / Journal of Biophysical Chemistry 3 (2012) 16-28 22 degree of substrate competitive inhibition was found (data not shown). Alternatively, at fixed inhibitory values of H2O2 con- centration, the v vs. [AH2] data analysis provided a series of alternative equations similar to Eqs.A13-A16. Accordingly, the corresponding plots of 1/v vs. 1/[AH2] at fixed [H2O2] are also linear and intersect on the y axis and the plots of K/V vs. [H2O2] should also be linear. This graphic behaviour was obtained for ferulic acid at fixed [H2O2] (Figure 5) and also for the rest of substrates studied (data not shown). Thus, competitive substrate inhibition was observed in the H2O2-assisted RPTP-catalyzed oxidation reactions for both substrates, as would be expected for a Ping- Pong reaction mechanism. The corresponding inhibition constants— and —obtained, for the case of ferulic acid, were 1.24 × 10–3 M and 2.7 × 10–5 M, re- spectively. 22 HO SI K2 AH SI K In light of the above, the combined initial-rate and substrate inhibition results exclude an ordered or random sequential reaction mechanism, and are only consistent with a Ping-Pong Bi Bi mechanism according to the no- tation of Cleland [29] as the minimal kinetic model for H2O2-assisted RPTP-catalyzed substrate oxidation reac- tions. Ping-Pong reaction kinetics has also been observed for several other peroxidase-catalyzed oxidations mediated by Compound I. The results of initial-rate studies of the hydrogen peroxide-supported oxidation of guaiacol by turnip peroxidase [53] and of the oxidation of ferrocyto- chrome c catalyzed by horseradish peroxidase [54] and yeast cytochrome c peroxidase [55] are consistent with a Ping-Pong mechanism. Initial-rate kinetic studies of the KN (mM·s) Figure 5. Primary double-reciprocal plot of the initial rate of ferulic acid oxidation as a function of ferulic acid con- centration at fixed hydrogen peroxide inhibitory concentra- tions (1.5 mM (■), 2.0 mM (○), 3.0 mM (●)). The inset shows the secondary plot of K/V vs. H2O2 concentration. See text for other experimental conditions. oxidation of ferrocytochrome c by Pseudomonas aerugi- nosa cytochrome c peroxidase yielded intersecting plots, which were initially interpreted as indicating a sequential reaction mechanism [56]. However, subsequent studies demonstrated that the intersecting plots arose from the formation of an inactive hydrogen peroxide-enzyme com- plex, and the mechanism of the reaction was reinter- preted to be of the modified Ping-Pong type [57]. It has recently been reported that Chamaerops excelsa palm tree peroxidase also exhibits a Ping-Pong Bi Bi mecha- nism for the H2O2-assisted catalyzed oxidation reactions of guaiacol, ABTS, o-dianisidine and o-phenylendiamine [48]. 3.5. Partitioning Ratio (r) for the Inactivation of RPTP by H2O2 Using plots of the percent residual activity for each substrate against the [peroxide]/[enzyme] ratio, the ratio required in each case for 100% inactivation can be ob- tained from the intercept of the fitted line. From this value, the partitioning ratio can be calculated using the following equation: 22 t R o HO A1 A1 A1rRPT P (4) where AR is the residual activity; At and Ao are the activi- ties at time (t) (end of the reaction) and zero respectively; (r) is the partitioning ratio or inactivation turnover num- ber (number of catalytic cycles given by enzymes before their inactivation), and [H2O2] and [RPTP] are the initial concentrations of H2O2 and enzyme [33]. Figure 6 shows the plots of the percent residual activ- ity against the [H2O2]/[RPTP] ratio for the guaiacol sub- strate. In light of the consumption of two moles of H2O2 in each catalytic cycle (one mole for the formation of Compound I and another for inactivation or catalysis) [58-60], the (r) value, calculated from the corresponding fitting of the data to the first linear section of the curve (Figure 6), was 2154 ± 100. 3.6. Kinetics of Inactivation by H2O2 In the absence of reducing substrate, the class I, II and III peroxidases studied to date undergo suicide inactiva- tion by H2O2. The inactivation kinetics shows profiles that differ for each enzyme. The inactivation kinetics of RPTP by hydrogen peroxide, at a range of different con- centrations, using guaiacol as co-substrate, is biphasic, with a rapid inactivation step in the first 10 - 15 min, followed by a longer-lasting, slow step (data not shown). The same biphasic behaviour has been reported for the inactivation by H2O2 of HRP [32,33,61] and melon per- oxidase [62]. Other peroxidases studied to date also Copyright © 2012 SciRes. OPEN ACCESS
 L. S. Zamorano et al. / Journal of Biophysical Chemistry 3 (2012) 16-28 23 Figure 6. Sensitivity to inactivation of RPTP at differ- ent [H2O2]/[RPT] molar ratios. RPTP was incubated with molar excesses of peroxide in universal buffer (30 mM, pH 6.5) and when the reaction was complete (24 h incubation), the percentage residual activities were measured with guaiacol at a fixed concentration of the enzyme (136 nM). exhibit different types of behaviour [33,48]. Then, the biphasic behaviour of data of residual per- oxidase activity versus time could be fitted to a sum of exponentials: ob ob t R Aae be k tk (5) Consequently, following Eq.6, a plot of the logarithms of the percentage residual activities (lnAR) against time afforded straight lines for each segment of time (data not shown), with slopes equivalent to the observed rate con- stants of the inactivation (kob, kob′) [38]. t ob o A ln t Ak (6) The inactivation of RPTP by H2O2 clearly showed saturation kinetics, as seen from the hyperbolic curves fitted to the plot of kob against [H2O2] for each segment of time (Figure 7). Then, the observed first-order rate constants of inacti- vation (kob) can be fitted to the following equation: app inact22 ob app I2 HO HO k kK 2 (7) where , a first-order inactivation rate constant, and app inact k app I , an inhibitor-binding constant, were obtained from the corresponding fitting of the data by a linear regres- sion model [63]. In the absence of reducing substrates and at high H2O2 concentrations, RPTP is inactivated in a time- and H2O2 concentration-dependent process, exhibiting suicide or mechanism-based inactivation kinetics. Similar types of behaviour have been reported by other authors [16,32,33, Figure 7. Biphasic inactivation kinetics of RPTP by H2O2. Plot of kob (first-order in- activation rate constant) against H2O2 con- centration for the first and rapid inactiva- tion step (A) and for the longer-lasting slow step (B). 61,64] in studies addressing H2O2-mediated inactivation under identical experimental conditions of temperature, pH, and the concentrations of peroxidase and H2O2. Nevertheless there are, differences in the shapes of the inactivation curves, in the magnitudes and rates of inac- tivation at specific H2O2 concentrations, and in the maximum rate of inactivation () as compared with the results reported here. app inact k The inactivation process involves the participation of two pathways, one reversible and other irreversible, which may or may not function independently of each other and whose individual contribution to the overall inactivation process seems to be dependent upon the H2O2 concentration. For enzyme inactivation, a second molecule of hydrogen peroxide would be required; not for the inactivation per se but for the formation of Com- pound III. Once Compound III has been formed, a frac- tion of that population would be transformed into an in- active species. Another interpretation would be that once a molecule of Compound III has been formed, it has a certain probability of decaying into an inactive species instead of decaying into an active one that would again become engaged in the catalytic cycle. In the case of Compound I, several authors [32,33,64] have suggested the existence of a partitioning between the two pathways. Their model, based on studies at high H2O2 concentrations, invokes a further partitioning be- tween these two inactivation pathways and suggests the existence of a catalytic reaction in which H2O2 would be consumed with relatively little harm to the enzyme [64]. The model is actually a simplification of the full ki- netic approach developed previously by Arnao et al., Copyright © 2012 SciRes. OPEN ACCES S
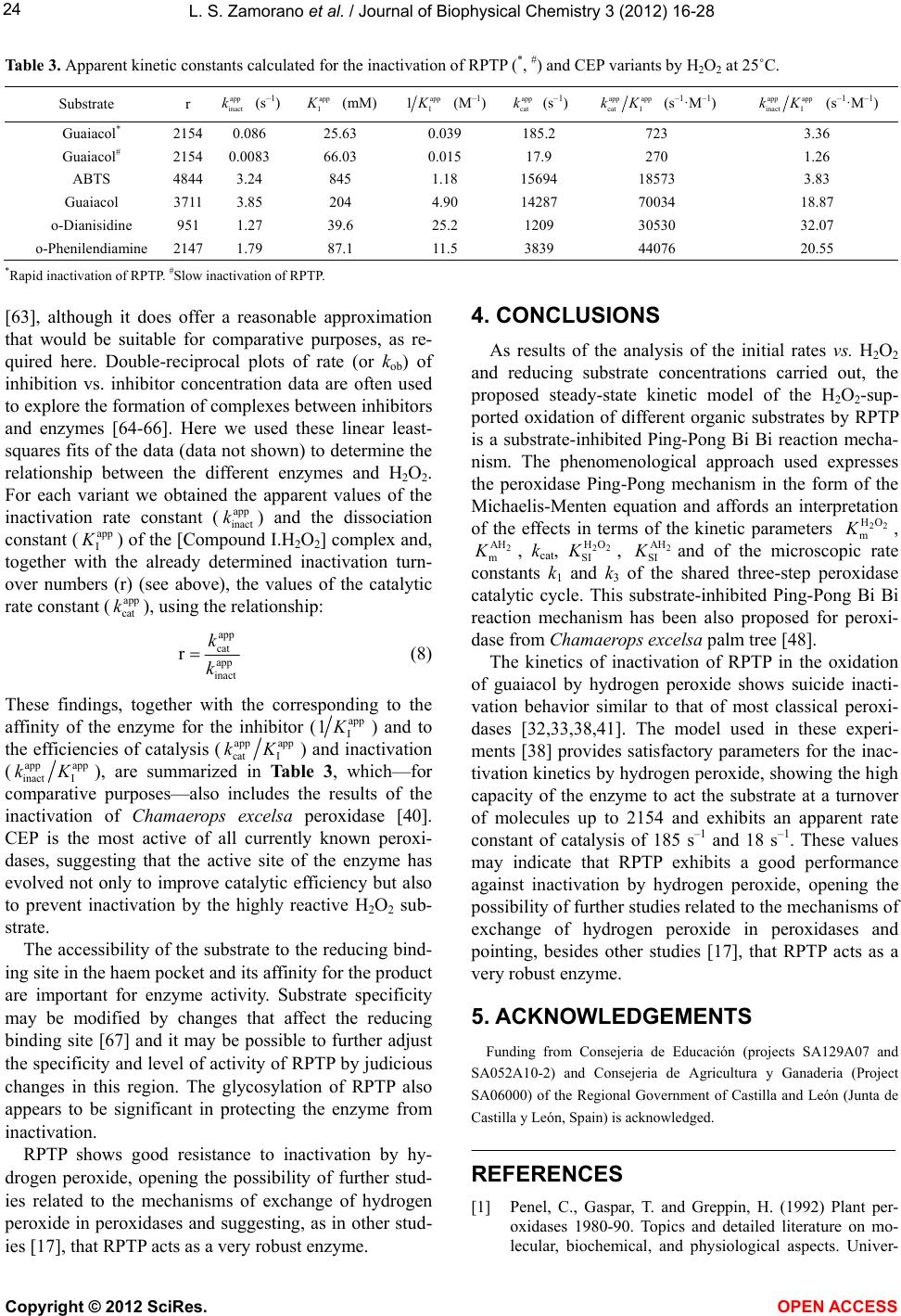 L. S. Zamorano et al. / Journal of Biophysical Chemistry 3 (2012) 16-28 Copyright © 2012 SciRes. 24 Table 3. Apparent kinetic constants calculated for the inactivation of RPTP (*, #) and CEP variants by H2O2 at 25˚C. Substrate r app inact k (s–1) app I (mM)app I 1 (M–1)app cat k (s–1)app app cat I kK (s–1·M–1) app app inactI kK (s–1·M–1) Guaiacol* 2154 0.086 25.63 0.039 185.2 723 3.36 Guaiacol# 2154 0.0083 66.03 0.015 17.9 270 1.26 ABTS 4844 3.24 845 1.18 15694 18573 3.83 Guaiacol 3711 3.85 204 4.90 14287 70034 18.87 o-Dianisidine 951 1.27 39.6 25.2 1209 30530 32.07 o-Phenilendiamine 2147 1.79 87.1 11.5 3839 44076 20.55 *Rapid inactivation of RPTP. #Slow inactivation of RPTP. 4. CONCLUSIONS [63], although it does offer a reasonable approximation that would be suitable for comparative purposes, as re- quired here. Double-reciprocal plots of rate (or kob) of inhibition vs. inhibitor concentration data are often used to explore the formation of complexes between inhibitors and enzymes [64-66]. Here we used these linear least- squares fits of the data (data not shown) to determine the relationship between the different enzymes and H2O2. For each variant we obtained the apparent values of the inactivation rate constant () and the dissociation constant ( app inact k app I ) of the [Compound I.H2O2] complex and, together with the already determined inactivation turn- over numbers (r) (see above), the values of the catalytic rate constant (), using the relationship: app cat k As results of the analysis of the initial rates vs. H2O2 and reducing substrate concentrations carried out, the proposed steady-state kinetic model of the H2O2-sup- ported oxidation of different organic substrates by RPTP is a substrate-inhibited Ping-Pong Bi Bi reaction mecha- nism. The phenomenological approach used expresses the peroxidase Ping-Pong mechanism in the form of the Michaelis-Menten equation and affords an interpretation of the effects in terms of the kinetic parameters , , kcat, , and of the microscopic rate constants k1 and k3 of the shared three-step peroxidase catalytic cycle. This substrate-inhibited Ping-Pong Bi Bi reaction mechanism has been also proposed for peroxi- dase from Chamaerops excelsa palm tree [48]. 22 HO m K 2 AH m K22 HO SI K2 AH SI K app cat app inact rk k (8) The kinetics of inactivation of RPTP in the oxidation of guaiacol by hydrogen peroxide shows suicide inacti- vation behavior similar to that of most classical peroxi- dases [32,33,38,41]. The model used in these experi- ments [38] provides satisfactory parameters for the inac- tivation kinetics by hydrogen peroxide, showing the high capacity of the enzyme to act the substrate at a turnover of molecules up to 2154 and exhibits an apparent rate constant of catalysis of 185 s–1 and 18 s–1. These values may indicate that RPTP exhibits a good performance against inactivation by hydrogen peroxide, opening the possibility of further studies related to the mechanisms of exchange of hydrogen peroxide in peroxidases and pointing, besides other studies [17], that RPTP acts as a very robust enzyme. These findings, together with the corresponding to the affinity of the enzyme for the inhibitor (app I 1K) and to the efficiencies of catalysis (appapp cat I kK) and inactivation (app app inact I kK), are summarized in Table 3, which—for comparative purposes—also includes the results of the inactivation of Chamaerops excelsa peroxidase [40]. CEP is the most active of all currently known peroxi- dases, suggesting that the active site of the enzyme has evolved not only to improve catalytic efficiency but also to prevent inactivation by the highly reactive H2O2 sub- strate. The accessibility of the substrate to the reducing bind- ing site in the haem pocket and its affinity for the product are important for enzyme activity. Substrate specificity may be modified by changes that affect the reducing binding site [67] and it may be possible to further adjust the specificity and level of activity of RPTP by judicious changes in this region. The glycosylation of RPTP also appears to be significant in protecting the enzyme from inactivation. 5. ACKNOWLEDGEMENTS Funding from Consejeria de Educación (projects SA129A07 and SA052A10-2) and Consejeria de Agricultura y Ganaderia (Project SA06000) of the Regional Government of Castilla and León (Junta de Castilla y León, Spain) is acknowledged. RPTP shows good resistance to inactivation by hy- drogen peroxide, opening the possibility of further stud- ies related to the mechanisms of exchange of hydrogen peroxide in peroxidases and suggesting, as in other stud- ies [17], that RPTP acts as a very robust enzyme. REFERENCES [1] Penel, C., Gaspar, T. and Greppin, H. (1992) Plant per- oxidases 1980-90. Topics and detailed literature on mo- lecular, biochemical, and physiological aspects. Univer- OPEN A CCESS
 L. S. Zamorano et al. / Journal of Biophysical Chemistry 3 (2012) 16-28 25 sity of Geneva, Geneva. [2] Dordick, J.S., Marletta, M.A. and Klibanov, A.M. (1987) Polymerization of phenols catalyzed by peroxidase in nonaqueous media. Biotechnology and Bioengineering, 30, 31-36. doi:10.1002/bit.260300106 [3] Akkara, J.A., Senecal, K.J. and Kaplan, D.L. (1991) Synthesis and characterization of polymers produced by horseradish peroxidase in dioxane. Journal of Polymer Science Part A: Polymer Chemist ry, 29, 1561-1574. doi:10.1002/pola.1991.080291105 [4] Thompson, R.Q. (1987) Peroxidase-based colorimetric determination of L-ascorbic acid. Analytical Chemistry, 59, 1119-1121. doi:10.1021/ac00135a011 [5] Weng, Z., Hendrickx, M., Maesmans, G. and Tobback, P. (1991) Thermostability of soluble and immobilized horse- radish peroxidase. Journal of Food Science, 56, 574-578. doi:10.1111/j.1365-2621.1991.tb05328.x [6] Arseguel, D. and Baboulène, M.J. (1994) Removal of Phenol from coupling of talc and peroxidase—Applica- tion for depollution of waste-water containing phenolic- compounds. Journal of Chemical Technology and Bio- technology, 61, 331-335. doi:10.1002/jctb.280610408 [7] Adler, P.R., Arora, R., El Ghaouth, A., Glenn, D.M. and Solar, J.M. (1994) Bioremediation of phenolic com- pounds from water with plant root surface peroxidases. Journal of Environmental Quality, 23, 1113-1117. doi:10.2134/jeq1994.00472425002300050038x [8] Zamorano, L.S., Roig, M.G., Villar, E. and Shnyrov, V.L. (2007) The versatile peroxidases. Current Topics of Bio- chemical Research, 9, 1-26. [9] Dunford, H.B. (1999) Heme peroxidases. John Wiley & Sons, Inc., New York. [10] Schuller, D.J., Ban, N., Van Huystee, R.B., McPherson, A. and Poulos, T.L. (1996) The crystal structure of peanut peroxidase. Structure, 4, 311-321. doi:10.1016/S0969-2126(96)00035-4 [11] Rasmussen, C.B., Hiner, A.N., Smith, A.T. and Welinder, K.G. (1998) Effect of calcium, other ions, and pH on the reactions of barley peroxidase with hydrogen peroxide and fluoride. Control of activity through conformational change. The Journal of Biological Chemistry, 273, 2232- 2240. doi:10.1074/jbc.273.4.2232 [12] Kvaratskhelia, M., Winkel, C. and Thorneley, R.N. (1997) Purification and characterization of a novel class III per- oxidase isoenzyme from tea leaves. Plant Physiology, 114, 1237-1245. doi:10.1104/pp.114.4.1237 [13] Ostergaard, L., Abelskov, A.K., Mattsson, O. and Welinder, K.G. (1996) Structure and organ specificity of an anionic peroxidase from Arabidopsis thaliana cell suspension culture. FEBS Letters, 398, 243-247. doi:10.1016/S0014-5793(96)01244-6 [14] Rodríguez, A., Pina, D.G., Yélamos, B., Castillo León, J.J., Zhadan, G.G., Villar, E., Gavilanes, F., Roig, M.G., Sakharov, I.Y. and Shnyrov, V.L. (2002) Thermal stability of peroxidase from the african oil palm tree Elaeis gui- neensis. European Journal of Biochemistry, 269, 2584- 2590. doi:10.1046/j.1432-1033.2002.02930.x [15] Watanabe, L., Nascimiento, A.S., Zamorano, L.S., Shny- rov, V.L. and Polikarpov, I. (2007) Purification, crystalli- zation and preliminary X-ray diffraction analysis of royal palm tree (Roystonea regia) peroxidase. Acta Crystallo- graphica Section F, 63, 780-783. [16] Zamorano, L.S., Pina, D.G., Arellano, J.B., Bursakov, S. A., Zhadan, A.P., Calvete, J.J., Sanz, L., Nielsen, P.R., Villar, E., Gavel, O., Roig, M.G., Watanabe, L., Polikarpov, I. and Shnyrov, V.L. (2008) Thermodynamic characteriza- tion of the palm tree Roystonea regia peroxidase stability. Biochimie, 90, 1737-1749. doi:10.1016/j.biochi.2008.07.010 [17] Zamorano, L.S., Vilarmau, S.B., Arellano, J.B., Zhandan, G.G., Hidalgo-Cuadrado, N., Bursakov, S.A., Roig, M.G. and Shnyrov, V.L. (2009) Thermal stability of peroxidase from Chamaerops excelsa palm tree at pH 3. International Journal of Biological Macromolecules, 44, 326-332. doi:10.1016/j.ijbiomac.2009.01.004 [18] Watanabe, L., Ribeiro de Moura, P., Bleicher, L., Nasci- miento, A.S., Zamorano, L.S., Calvete, J.J., Sanz, L., Pérez, A., Bursakov, S., Roig, M.G., Shnyrov, V.L. and Polikarpov, I. (2009) Crystal structure and statistical cou- pling analysis of highly glycosylated peroxidase from royal palm tree (Roystonea regia). Journal of Structural Biology, 169, 226-242. doi:10.1016/j.jsb.2009.10.009 [19] Poulos, T.L. and Kraut, J. (1980) The stereochemistry of peroxidase catalysis. The Journal of Biological Chemistry, 255, 8199-8205. [20] Rasmussen, C.B., Dunford, H.B. and Welinder, K.G. (1995) Rate enhancement of compound I formation of barley peroxidase by ferulic acid, caffeine acid and coni- feryl alcohol. Biochemistry, 34, 4022-4029. doi:10.1021/bi00012a021 [21] Rodriguez-López, J.N., Lowe, D.L., Hernández-Ruiz, J., Hiner, A.N.P., Garcia-Cánovas, F. and Thorneley, R.N.F. (2001) Mechanism of reaction of hydrogen peroxide with horseradish peroxidase: Identification of intermediates in the catalytic cycle. Journal of the American Chemical Society, 123, 11838-11847. doi:10.1021/ja011853+ [22] Dunford, H.B. and Stillman, J.S. (1976) On the function and mechanism of action of peroxidases. Coordination Chemitry Reviews, 19, 187-251. doi:10.1016/S0010-8545(00)80316-1 [23] Wang, W., Noël, S., Desmadril, M., Guéguen, J. and Mi- chons, T. (1999) Kinetic evidence for the formation of Michaelis-Menten like complex between horseradish pe- roxidase Compound II and di-(N-acetyl-L-tyrosine). Bio- chemical Journal, 340, 329-336. doi:10.1042/0264-6021:3400329 [24] Childs, R.E. and Bardsley, W.G. (1975) The steady-state kinetics of peroxidase with 2,2’-azino-di-(3-ethyl-benthi- azoline-6-sulphonic acid) as chromogen. Bioche mical Jour- nal, 14 5, 93-103. [25] Wolters, G., Kuijpers, L., Kacaki, J. and Schuurs, A. (1976) Solid-phase enzyme-immunoassay for detection of hepa- titis B surface antigen. Journal of Clinical Pathology, 29, 873-879. doi:10.1136/jcp.29.10.873 [26] Bovaird, J.H., Ngo, T.T. and Lenhoff, H.M. (1982) Opti- mizing the o-phenylendiamine assay for horseradish per- oxidase: Effects of phosphate and pH, substrate and en- Copyright © 2012 SciRes. OPEN ACCES S
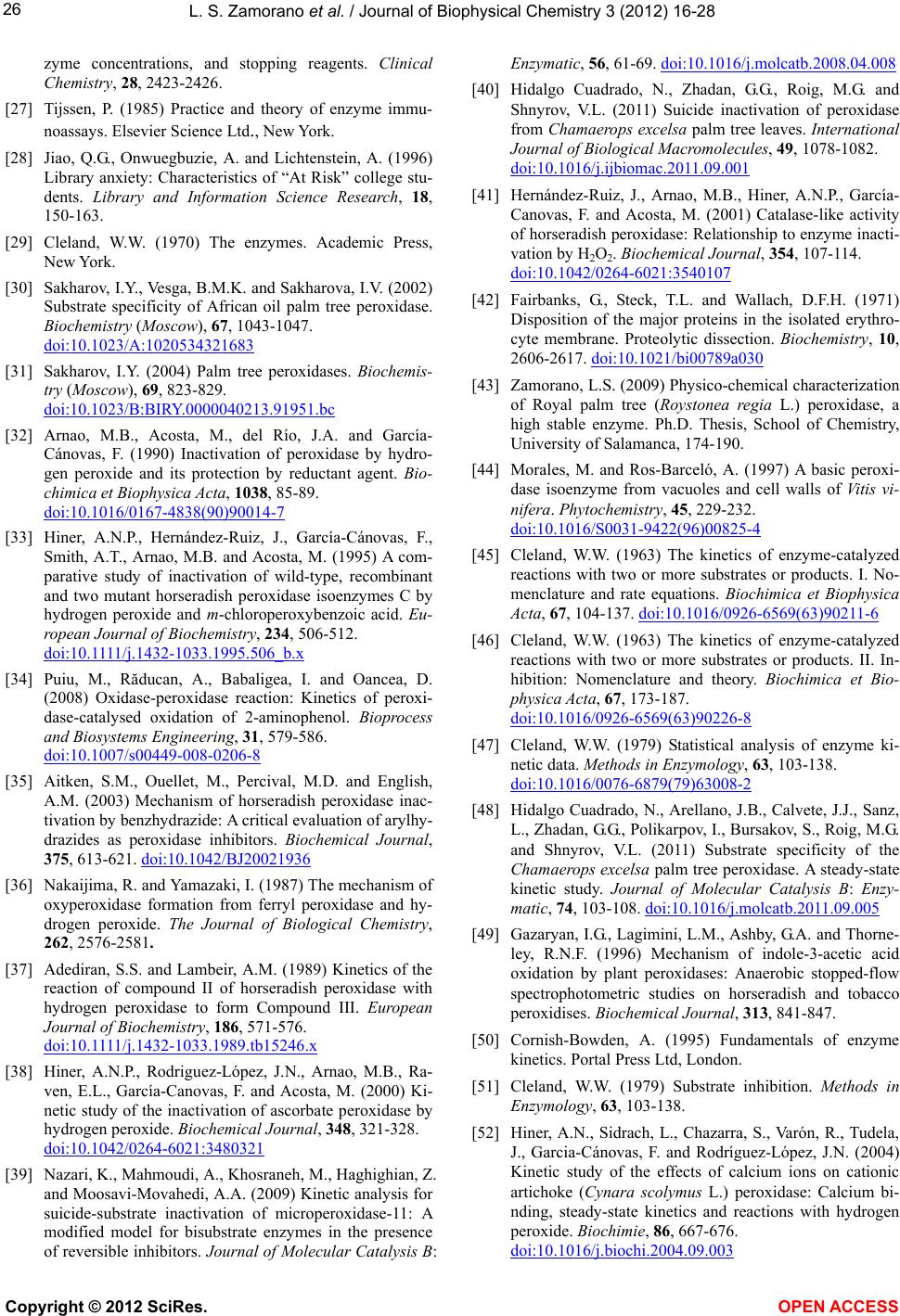 L. S. Zamorano et al. / Journal of Biophysical Chemistry 3 (2012) 16-28 26 zyme concentrations, and stopping reagents. Clinical Chemistry, 28, 2423-2426. [27] Tijssen, P. (1985) Practice and theory of enzyme immu- noassays. Elsevier Science Ltd., New York. [28] Jiao, Q.G., Onwuegbuzie, A. and Lichtenstein, A. (1996) Library anxiety: Characteristics of “At Risk” college stu- dents. Library and Information Science Research, 18, 150-163. [29] Cleland, W.W. (1970) The enzymes. Academic Press, New York. [30] Sakharov, I.Y., Vesga, B.M.K. and Sakharova, I.V. (2002) Substrate specificity of African oil palm tree peroxidase. Biochemistry (Moscow), 67, 1043-1047. doi:10.1023/A:1020534321683 [31] Sakharov, I.Y. (2004) Palm tree peroxidases. Biochemis- try (Moscow), 69, 823-829. doi:10.1023/B:BIRY.0000040213.91951.bc [32] Arnao, M.B., Acosta, M., del Río, J.A. and García- Cánovas, F. (1990) Inactivation of peroxidase by hydro- gen peroxide and its protection by reductant agent. Bio- chimica et Biophysica Acta, 1038, 85-89. doi:10.1016/0167-4838(90)90014-7 [33] Hiner, A.N.P., Hernández-Ruiz, J., García-Cánovas, F., Smith, A.T., Arnao, M.B. and Acosta, M. (1995) A com- parative study of inactivation of wild-type, recombinant and two mutant horseradish peroxidase isoenzymes C by hydrogen peroxide and m-chloroperoxybenzoic acid. Eu- ropean Journal of Biochemistry, 234, 506-512. doi:10.1111/j.1432-1033.1995.506_b.x [34] Puiu, M., Răducan, A., Babaligea, I. and Oancea, D. (2008) Oxidase-peroxidase reaction: Kinetics of peroxi- dase-catalysed oxidation of 2-aminophenol. Bioprocess and Biosystems Engineering, 31, 579-586. doi:10.1007/s00449-008-0206-8 [35] Aitken, S.M., Ouellet, M., Percival, M.D. and English, A.M. (2003) Mechanism of horseradish peroxidase inac- tivation by benzhydrazide: A critical evaluation of arylhy- drazides as peroxidase inhibitors. Biochemical Journal, 375, 613-621. doi:10.1042/BJ20021936 [36] Nakaijima, R. and Yamazaki, I. (1987) The mechanism of oxyperoxidase formation from ferryl peroxidase and hy- drogen peroxide. The Journal of Biological Chemistry, 262, 2576-2581. [37] Adediran, S.S. and Lambeir, A.M. (1989) Kinetics of the reaction of compound II of horseradish peroxidase with hydrogen peroxidase to form Compound III. European Journal of Biochemistry, 186, 571-576. doi:10.1111/j.1432-1033.1989.tb15246.x [38] Hiner, A.N.P., Rodriguez-López, J.N., Arnao, M.B., Ra- ven, E.L., García-Canovas, F. and Acosta, M. (2000) Ki- netic study of the inactivation of ascorbate peroxidase by hydrogen peroxide. Biochemical Journal, 348, 321-328. doi:10.1042/0264-6021:3480321 [39] Nazari, K., Mahmoudi, A., Khosraneh, M., Haghighian, Z. and Moosavi-Movahedi, A.A. (2009) Kinetic analysis for suicide-substrate inactivation of microperoxidase-11: A modified model for bisubstrate enzymes in the presence of reversible inhibitors. Journal of Molecular Catalysis B: Enzymatic, 56, 61-69. doi:10.1016/j.molcatb.2008.04.008 [40] Hidalgo Cuadrado, N., Zhadan, G.G., Roig, M.G. and Shnyrov, V.L. (2011) Suicide inactivation of peroxidase from Chamaerops excelsa palm tree leaves. International Journal of Biological Macromolecules, 49, 1078-1082. doi:10.1016/j.ijbiomac.2011.09.001 [41] Hernández-Ruiz, J., Arnao, M.B., Hiner, A.N.P., García- Canovas, F. and Acosta, M. (2001) Catalase-like activity of horseradish peroxidase: Relationship to enzyme inacti- vation by H2O2. Biochemical Journal, 354, 107-114. doi:10.1042/0264-6021:3540107 [42] Fairbanks, G., Steck, T.L. and Wallach, D.F.H. (1971) Disposition of the major proteins in the isolated erythro- cyte membrane. Proteolytic dissection. Biochemistry, 10, 2606-2617. doi:10.1021/bi00789a030 [43] Zamorano, L.S. (2009) Physico-chemical characterization of Royal palm tree (Roystonea regia L.) peroxidase, a high stable enzyme. Ph.D. Thesis, School of Chemistry, University of Salamanca, 174-190. [44] Morales, M. and Ros-Barceló, A. (1997) A basic peroxi- dase isoenzyme from vacuoles and cell walls of Vitis vi- nifera. Phytochemistry, 45, 229-232. doi:10.1016/S0031-9422(96)00825-4 [45] Cleland, W.W. (1963) The kinetics of enzyme-catalyzed reactions with two or more substrates or products. I. No- menclature and rate equations. Biochimica et Biophysica Acta, 67, 104-137. doi:10.1016/0926-6569(63)90211-6 [46] Cleland, W.W. (1963) The kinetics of enzyme-catalyzed reactions with two or more substrates or products. II. In- hibition: Nomenclature and theory. Biochimica et Bio- physica Acta, 67, 173-187. doi:10.1016/0926-6569(63)90226-8 [47] Cleland, W.W. (1979) Statistical analysis of enzyme ki- netic data. Methods in Enzymology, 63, 103-138. doi:10.1016/0076-6879(79)63008-2 [48] Hidalgo Cuadrado, N., Arellano, J.B., Calvete, J.J., Sanz, L., Zhadan, G.G., Polikarpov, I., Bursakov, S., Roig, M.G. and Shnyrov, V.L. (2011) Substrate specificity of the Chamaerops excelsa palm tree peroxidase. A steady-state kinetic study. Journal of Molecular Catalysis B: Enzy- matic, 74, 103-108. doi:10.1016/j.molcatb.2011.09.005 [49] Gazaryan, I.G., Lagimini, L.M., Ashby, G.A. and Thorne- ley, R.N.F. (1996) Mechanism of indole-3-acetic acid oxidation by plant peroxidases: Anaerobic stopped-flow spectrophotometric studies on horseradish and tobacco peroxidises. Biochemica l Jou rnal, 313, 841-847. [50] Cornish-Bowden, A. (1995) Fundamentals of enzyme kinetics. Portal Press Ltd, London. [51] Cleland, W.W. (1979) Substrate inhibition. Methods in Enzymology, 63, 103-138. [52] Hiner, A.N., Sidrach, L., Chazarra, S., Varón, R., Tudela, J., Garcia-Cánovas, F. and Rodríguez-López, J.N. (2004) Kinetic study of the effects of calcium ions on cationic artichoke (Cynara scolymus L.) peroxidase: Calcium bi- nding, steady-state kinetics and reactions with hydrogen peroxide. Biochimie, 86, 667-676. doi:10.1016/j.biochi.2004.09.003 Copyright © 2012 SciRes. OPEN ACCESS
 L. S. Zamorano et al. / Journal of Biophysical Chemistry 3 (2012) 16-28 Copyright © 2012 SciRes. 27 [53] Hosoya, T. (1960) Turnip peroxidase: I. Purification and physicochemical properties of multiple components in turnip peroxidise. The Journal of Biochemistry, 47, 369- 381. [54] Santimone, M. (1975) The mechanism of ferrocytochrome C oxidation by a horseradish isoperoxidase. Biochimie, 57, 91-96. doi:10.1016/S0300-9084(75)80114-3 OPEN A CCESS [55] Yonetani, T. and Ray, G.S. (1966) Studies on cytochrome c peroxidase. 3. Kinetics of the peroxidasic oxidation of ferrocytochrome c catalyzed by cytochrome c peroxidise. The Journal of Biological Chemistry, 241, 700-706. [56] Ronnberg, M. and Ellfolk, N. (1975) Pseudomonas cyto- chrome c peroxidase XI. Kinetics of the peroxidatic oxi- dation of Pseudomonas respiratory chain components. Acta Chemica Scandinavica Series B, 29, 719-727. doi:10.3891/acta.chem.scand.29b-0719 [57] Ronnberg, M., Araiso, T., Ellfolk, N. and Dunford, H.B. (1981) The reaction between reduced azurin and oxidized cytochrome c peroxidase from Pseudomonas aeruginosa. The Journal of Biological Chemistry, 256, 2471-2474. [58] Wariishi, H. and Gold, M.H. (1990) Lignin peroxidase Compound III. Mechanism of formation and decomposi- tion. The Journal of Biological Chemistry, 265, 2070- 2077. [59] Wariishi, H., Akikeswaran, L. and Gold, M.H. (1988) Manganese peroxidase from the basidiomycete Phanero- chaete chrysosporium: Spectral characterization of the oxidized states and the catalytic cycle. Biochemistry, 27, 5365-5370. doi:10.1021/bi00414a061 [60] Timofeevski, S.L., Reading, N.S. and Aust, S.D. (1998) Mechanisms for protection against inactivation of man- ganese peroxidase by hydrogen peroxide. Archives of Biochemistry and Biophysics, 356, 287-295. doi:10.1006/abbi.1998.0776 [61] Hiner, A.N., Hernandez-Ruíz, J., Arnao, M.B., García- Canovas, F. and Acosta, M. (1996) A comparative study of the purity, enzyme activity, and inactivation by hydro- gen peroxide of commercially available horseradish per- oxidase isoenzymes A and C. Biotechnology and Bioen- gineering, 50, 655-662. doi:10.1002/(SICI)1097-0290(19960620)50:6<655::AID- BIT6>3.0.CO;2-J [62] Rodriguez-Lopez, J.N., Espin, J.C., Del Amor, F., Tudela, J., Martinez, V., Cerdá, A. and Garcia-Cánovas, F. (2000) Purification and kinetic characterization of an anionic peroxidase from melon (Cucumis melo L.) cultivated un- der different salinity conditions. Journal of Agricultural and Food Chemistry, 48, 1537-1541. doi:10.1021/jf9905774 [63] Arnao, M.B., Acosta, M., del Río, J.A., Varón, R. and García-Cánovas, F. (1990) A kinetic study on the suicide inactivation of peroxidase by hydrogen peroxide. Bio- chimica et Biophysisca Acta, 1041, 43-47. [64] Bayton, K.J., Bewtra, J.K., Biswas, N. and Taylor, K.E. (1994) Inactivation of horseradish peroxidase by phenol and hydrogen peroxide: A kinetic investigation. Bio- chimica et Biophysica Acta, 1206, 272-278. doi:10.1016/0167-4838(94)90218-6 [65] Walsh, C.T. (1978) Enzymatic reaction mechanisms. Free- man Inc., San Francisco. [66] Ator, M.A., David, S.K. and Ortiz de Montellano, P.R. (1987) Protein control of prosthetic heme reactivity. Re- action of substrates with the heme edge of horseradish peroxidise. The Journal of Biological Chemistry, 262, 14954-14960. [67] Smith, A,T., Sanders, S.A., Thorneley, R.N.F., Burke, J.F. and Bray, R.R.C. (1992) Characterisation of a haem ac- tive-site mutant of horseradish peroxidase, Phe41-Val, with altered reactivity towards hydrogen peroxide and reducing substrates. European Journal of Biochemistry, 207, 507-519. doi:10.1111/j.1432-1033.1992.tb17077.x APPENDIX where V and K parameters are as follows: 2 max AH m2 V V1A K The rate equation for an enzyme-catalyzed Ping-Pong reaction with two substrates, H2O2 and AH2, in the ab- sence of products and at non-inhibitory substrate con- centrations, is given by H (A3) 22 2 HO m AH m2 1A K KK H (A4) 22 2 max2 22 HO AH m2m2222 VHOAH vAHH OH OAHKK 2 (A1) Thus plots of 1/v vs. 1/[H2O2] are linear and parallel at different fixed [AH2] since: which can be cast in the form of a rectangular hyperbola for fixed values of [AH2]: 22 11 vVVHO K (A5) 22 22 VHO vHO K (A2) Furthermore, the reciprocals of Eqs.A3 and A4 are
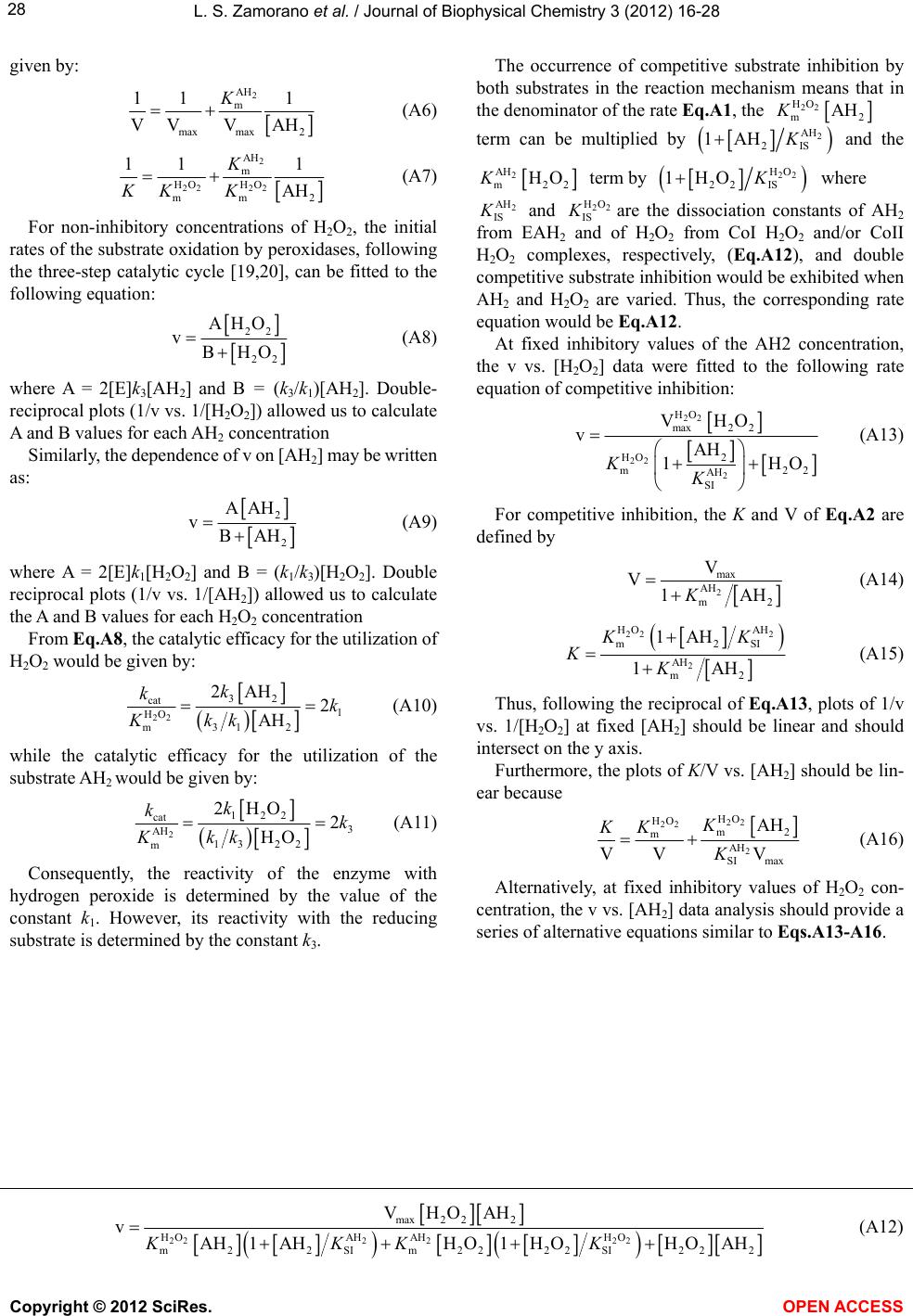 L. S. Zamorano et al. / Journal of Biophysical Chemistry 3 (2012) 16-28 28 given by: 2 AH m max max2 11 1 VVV AH K (A6) 2 22 22 AH m HO HO 2 mm 11 1 AH K KKK (A7) For non-inhibitory concentrations of H2O2, the initial rates of the substrate oxidation by peroxidases, following the three-step catalytic cycle [19,20], can be fitted to the following equation: 22 22 AHO vBHO (A8) where A = 2[E]k3[AH2] and B = (k3/k1)[AH2]. Double- reciprocal plots (1/v vs. 1/[H2O2]) allowed us to calculate A and B values for each AH2 concentration Similarly, the dependence of v on [AH2] may be written as: 2 2 AAH vBAH (A9) where A = 2[E]k1[H2O2] and B = (k1/k3)[H2O2]. Double reciprocal plots (1/v vs. 1/[AH2]) allowed us to calculate the A and B values for each H2O2 concentration From Eq.A8, the catalytic efficacy for the utilization of H2O2 would be given by: 22 32 cat 1 HO 31 2 m 2AH 2 AH k kk kk K (A10) while the catalytic efficacy for the utilization of the substrate AH2 would be given by: 2 122 cat 3 AH 13 22 m 2HO2 HO k kk kk K (A11) Consequently, the reactivity of the enzyme with hydrogen peroxide is determined by the value of the constant k1. However, its reactivity with the reducing substrate is determined by the constant k3. The occurrence of competitive substrate inhibition by both substrates in the reaction mechanism means that in the denominator of the rate Eq.A1, the 22 HO m2 AHK term can be multiplied by 2 AH K 2I S 1AH and the 2 AH m2 HOK2 term by 22 HO 22 IS 1HO K where 2 KAH IS and are the dissociation constants of AH2 from EAH2 and of H2O2 from CoI H2O2 and/or CoII H2O2 complexes, respectively, (Eq.A12), and double competitive substrate inhibition would be exhibited when AH2 and H2O2 are varied. Thus, the corresponding rate equation would be Eq.A12. 22 HO IS K At fixed inhibitory values of the AH2 concentration, the v vs. [H2O2] data were fitted to the following rate equation of competitive inhibition: 22 22 2 HO max2 2 2 HO m2 AH SI VHO vAH 1HKK 2 O (A13) For competitive inhibition, the K and V of Eq.A2 are defined by 2 max AH m2 V V1AK H (A14) 22 2 2 HO AH m2 AH m2 1AH 1AH KK KK SI (A15) Thus, following the reciprocal of Eq.A13, plots of 1/v vs. 1/[H2O2] at fixed [AH2] should be linear and should intersect on the y axis. Furthermore, the plots of K/V vs. [AH2] should be lin- ear because 22 22 2 HO HO m m AH SI max AH VV V K K K K 2 (A16) Alternatively, at fixed inhibitory values of H2O2 con- centration, the v vs. [AH2] data analysis should provide a series of alternative equations similar to Eqs.A13- A16. 222 222 max2 22 H OAHAHH O m22SIm2222SI222 VHOAH vAH1AHH O1HOH OAHKKK K (A12) Copyright © 2012 SciRes. OPEN ACCESS
|