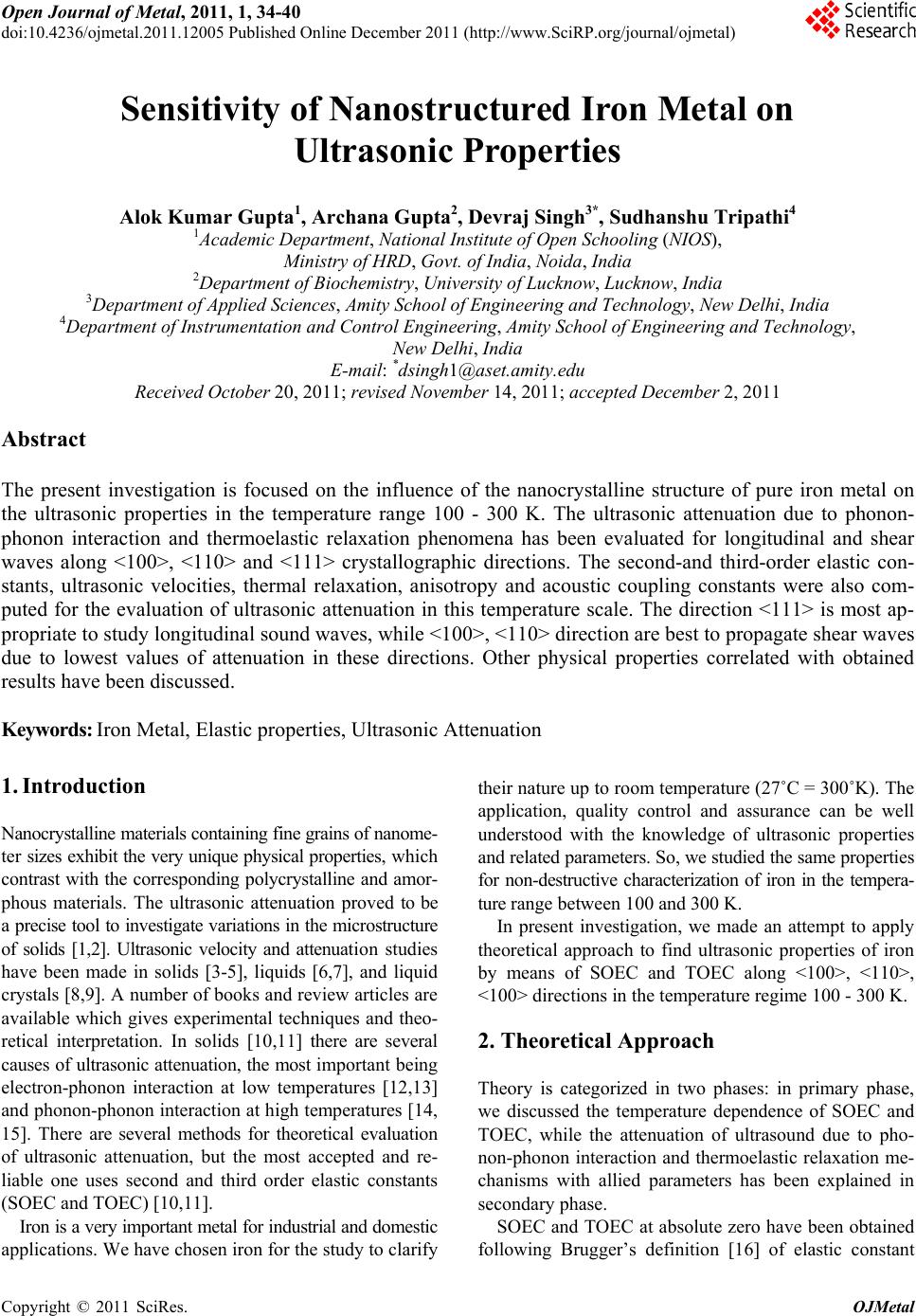 Open Journal of Metal, 2011, 1, 34-40 doi:10.4236/ojmetal.2011.12005 Published Online December 2011 (http://www.SciRP.org/journal/ojmetal) Copyright © 2011 SciRes. OJMetal Sensitivity of Nanostructured Iron Metal on Ultrasonic Properties Alok Kumar Gupta1, Archana Gupta2, Devraj Singh3*, Sudhanshu Tripathi4 1Academic Department, National Institute of Open Schooling (NIOS), Ministry of HRD, Govt. of India, Noida, India 2Department of Biochemistry, University of Lucknow, Lucknow, India 3Department of Applied Sciences, Amity School of Engineering and Technology, New Delhi, India 4Department of Instrumentation and Control Engineering, Amity School of Engineering and Technology, New Delhi, India E-mail: *dsingh1@aset.amity.edu Received October 20, 2011; revised November 14, 2011; accepted December 2, 2011 Abstract The present investigation is focused on the influence of the nanocrystalline structure of pure iron metal on the ultrasonic properties in the temperature range 100 - 300 K. The ultrasonic attenuation due to phonon- phonon interaction and thermoelastic relaxation phenomena has been evaluated for longitudinal and shear waves along <100>, <110> and <111> crystallographic directions. The second-and third-order elastic con- stants, ultrasonic velocities, thermal relaxation, anisotropy and acoustic coupling constants were also com- puted for the evaluation of ultrasonic attenuation in this temperature scale. The direction <111> is most ap- propriate to study longitudinal sound waves, while <100>, <110> direction are best to propagate shear waves due to lowest values of attenuation in these directions. Other physical properties correlated with obtained results have been discussed. Keywords: Iron Metal, Elastic properties, Ultrasonic Attenuation 1. Introduction Nanocrystalline materials containing fine grains of nanome- ter sizes exhibit the very unique physical properties, which contrast with the corresponding polycrystalline and amor- phous materials. The ultrasonic attenuation proved to be a precise tool to investigate variations in the microstructure of solids [1,2]. Ultrasonic velocity and attenuation studies have been made in solids [3-5], liquids [6,7], and liquid crystals [8,9]. A number of books and review articles are available which gives experimental techniques and theo- retical interpretation. In solids [10,11] there are several causes of ultrasonic attenuation, the most important being electron-phonon interaction at low temperatures [12,13] and phonon-phonon interaction at high temperatures [14, 15]. There are several methods for theoretical evaluation of ultrasonic attenuation, but the most accepted and re- liable one uses second and third order elastic constants (SOEC and TOEC) [10,11]. Iron is a very important metal for industrial and domestic applications. We have chosen iron for the study to clarify their nature up to room temperature (27˚C = 300˚K). The application, quality control and assurance can be well understood with the knowledge of ultrasonic properties and related parameters. So, we studied the same properties for non-destructive characterization of iron in the tempera- ture range between 100 and 300 K. In present investigation, we made an attempt to apply theoretical approach to find ultrasonic properties of iron by means of SOEC and TOEC along <100>, <110>, <100> directions in the temperature regime 100 - 300 K. 2. Theoretical Approach Theory is categorized in two phases: in primary phase, we discussed the temperature dependence of SOEC and TOEC, while the attenuation of ultrasound due to pho- non-phonon interaction and thermoelastic relaxation me- chanisms with allied parameters has been explained in secondary phase. SOEC and TOEC at absolute zero have been obtained following Brugger’s definition [16] of elastic constant
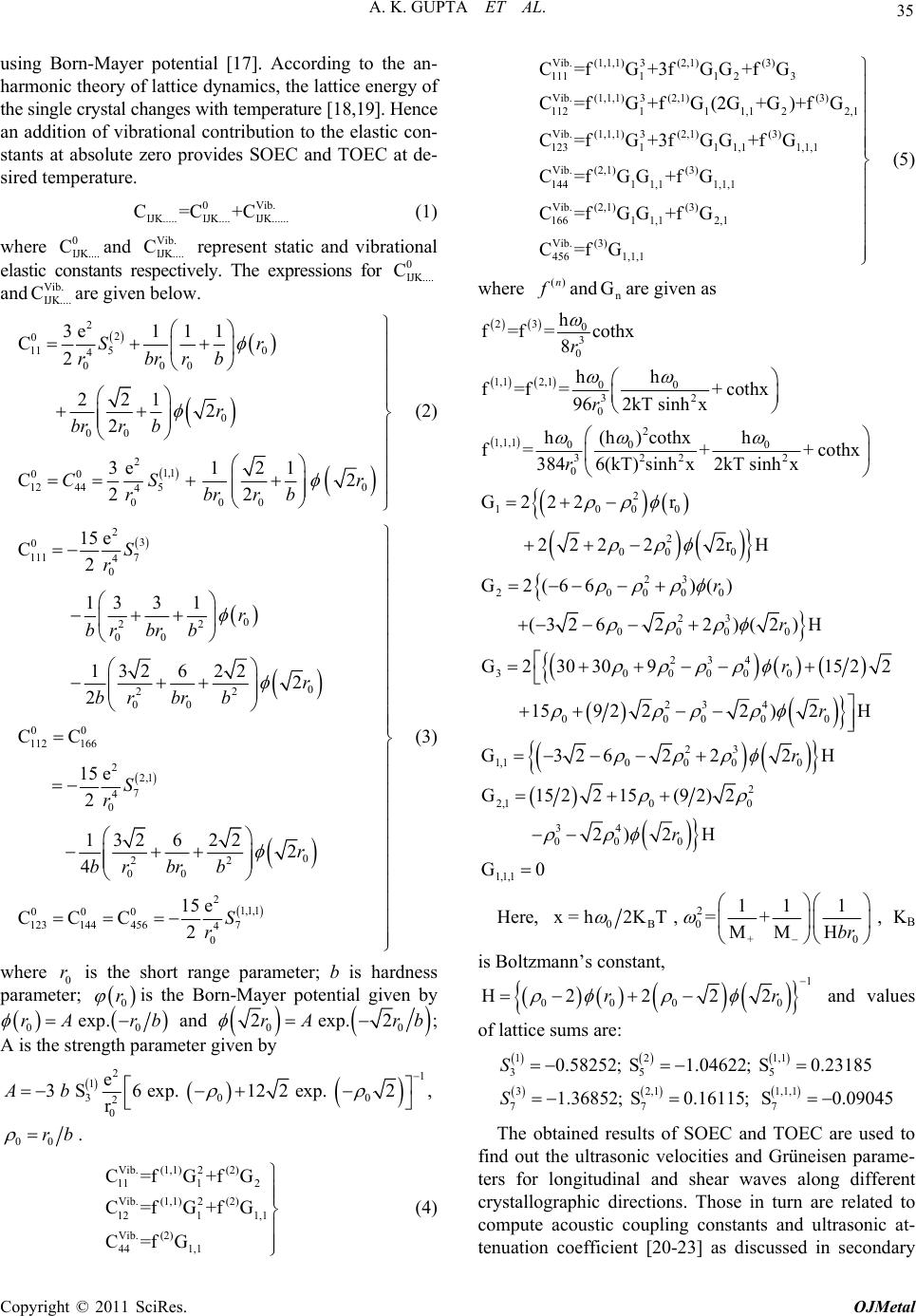 A. K. GUPTA ET AL. 35 . using Born-Mayer potential [17]. According to the an- harmonic theory of lattice dynamics, the lattice energy of the single crystal changes with temperature [18,19]. Hence an addition of vibrational contribution to the elastic con- stants at absolute zero provides SOEC and TOEC at de- sired temperature. 0Vib IJK..... IJK.... IJK...... C=C+C (1) where and represent static and vibrational elastic constants respectively. The expressions for and Care given below. 0 IJK.... C b. K.... Vib. IJK.... C 0 IJK.... C Vi IJ 22 0 11 50 4 000 0 00 21,1 00 12 4450 4 00 0 3e11 1 C2 221 2 2 3e12 1 C2 22 Sr br rbr r brr b CS r brr br (2) 23 0 111 7 4 0 0 22 0 0 0 22 0 0 00 112 166 22,1 7 4 0 0 22 0 0 000 123 144456 15 e C2 1331 1326 22 2 2 CC 15 e 2 1326 22 2 4 CCC S r r bbr rb r bbrrb S r r bbrrb 21,1,1 7 4 0 15 e 2S r (3) where 0 is the short range parameter; b is hardness parameter; is the Born-Mayer potential given by r 0 r exp. rb 00 rA and 0 2 exp.2rA rb 0 ; A is the strength parameter given by 21 1 30 0 2 0 e 3 S6 exp. 122 exp. 2 r Ab , 00 rb . Vib.(1,1) 2(2) 111 2 Vib.(1,1) 2(2) 121 1,1 Vib. (2) 44 1,1 C=f G+fG C=f G+fG C=fG (4) Vib.(1,1,1) 3(2,1)(3) 11111 23 Vib.(1,1,1) 3(2,1)(3) 112111,1 22,1 Vib.(1,1,1) 3(2,1)(3) 123111,11,1,1 Vib. (2,1)(3) 14411,11,1,1 Vib. (2,1)(3) 16611,12,1 Vi 456 C=fG+3f GG+fG C=fG+fG (2G+G)+fG C=fG+3f GG+fG C=f GG+fG C=f GG+fG Cb. (3) 1,1,1 =f G (5) where ()n and are given as n G 23 0 3 0 1,1 2,100 32 0 2 1,1,1 00 0 322 2 0 h f=f= cothx 8 hh f=f=+ cothx 962kT sinhx h(h)cothx h f=+ +cothx 3846(kT)sinhx2kT sinhx r r r 2 1000 2 00 0 G222 r 22222rH 23 20000 23 000 0 G2(66)() (32622)(2)H r r 234 300000 23 4 00000 G23030915 22 159222)2H r r 23 1100 00 G32622 2r ,H 2 2,10 0 34 00 0 G152215(92)2 2)2Hr 1,1,1 G0 Here, 0B x=h2KT ,2 0 +0 11 1 =+ MM Hbr , KB is Boltzmann’s constant, 1 000 0 H2222rr and values of lattice sums are: 121,1 35 5 32,1 1,1,1 777 0.58252; S1.04622; S0.23185 1.36852; S0.16115; S0.09045 S S The obtained results of SOEC and TOEC are used to find out the ultrasonic velocities and Grüneisen parame- ters for longitudinal and shear waves along different crystallographic directions. Those in turn are related to compute acoustic coupling constants and ultrasonic at- tenuation coefficient [20-23] as discussed in secondary Copyright © 2011 SciRes. OJMetal
 A. K. GUPTA ET AL. Copyright © 2011 SciRes. OJMetal 36 single crystal structure. The X-ray diffraction (XRD) pat- terns of iron nanowire arrays specified that most of the iron nanowire arrays have the obvious preferred orienta- tion along the <200> direction [28]. phase of theory and discussion section. The secondary objective of the present investigation is to develop a theory for evaluation of ultrasonic attenua- tion. Mason-Bateman Theory [11,14,15,19-27] is still widely used successfully to study the ultrasonic attenua- tion at higher temperature (300 K) in solids. It is more reliable theory to study anharmonicity of the crystals as it involves elastic constants directly through acoustic cou- pling constant “D” in the evaluation of ultrasonic atte- nuation (α). The thermoelastic loss and Akhiezer loss [24] under condition 1 is given by: The ultrasonic attenuation due to different mechanism at high temperatures are evaluated using nearest neighbour distance (r0) = 1.24 Å and Born-Mayer (hardness) pa- rameter (b) = 0.303 Å for Fe. The SOEC and TOEC are calculated at different temperatures. The values of den- sity are taken from the literature [29]. The microstructure of Fe was tested by transmission electron microscopy and X-ray diffraction. The mean size was found between 100 and 200 nm [30]. 2 2j 5 th iL =kT2V (6) The computed values of SOEC and TOEC at high temperatures are listed in Table 1. It is clear from Table 1 that, out of nine elastic constants, five (i.e., C11, C44, C111, C 166 and C144) are increasing and three (i.e., C12, C112, and C123) are decreasing with the temperature while C456 is found to be unaffected. The increase or decrease in elastic constants is mainly due to two basic parameters i.e., lattice parameter and hardness parameter with other common parameters as shown in Equations (1)-(5). 2 Akh 0 =ED (6V) 3 (7) where is the density; is angular frequency of ul- trasonic wave; k is the thermal conductivity; E0 is the thermal energy; V is velocity of longitudinal and shear waves; T is the temperature in Kelvin scale; L and S represent longitudinal and shear waves. Grüneisen num- ber i j (i is the mode of propagation and j is the direc- tion of propagation) is related to SOEC and TOEC. The thermal relaxation time for longitudinal wave is twice that of shear wave. This type of behaviour has already been found in ma- terials like Ce, Yb and Th [31]. Figure 1 shows that the 2 LS VD =0.5== 3k C V (8) where CV is the specific heat per unit volume and VD is the Debye average velocity. The acoustic coupling con- stant, which is measure of conversion of ultrasonic en- ergy into thermal energy, can be obtained by: 22 jj iiV D=93 γCT E 0 (9) 3. Results and Discussion The Transmission Electron Microscope (TEM) shows that the iron nanowires are highly uniform and exhibit a Figure 1. Temperature vs. C44/C12. Table 1. SOEC and TOEC (1011 N/m2) of Fe-metal in the temperature range 100 - 300 K. Temp.[K] C11 C 12 C 44 C 111 C 112 C 123 C 144 C 166 C 456 100 1.8261 0.6918 0.5682 –20.6498 –0.7016 0.4734 0.4785 –0.7376 0.4441 150 1.8270 0.6903 0.5697 –20.5068 –0.6564 0.4964 0.5042 –0.7104 0.4526 200 1.8280 0.6887 0.5712 –20.3637 –0.6112 0.5195 0.5299 –0.6832 0.4611 250 1.8289 0.6872 0.5727 –20.2206 –0.5659 0.5426 0.5555 –0.6560 0.4695 300 1.8299 0.6856 0.5742 –20.0776 –0.5207 0.5657 0.5812 –0.6288 0.4780
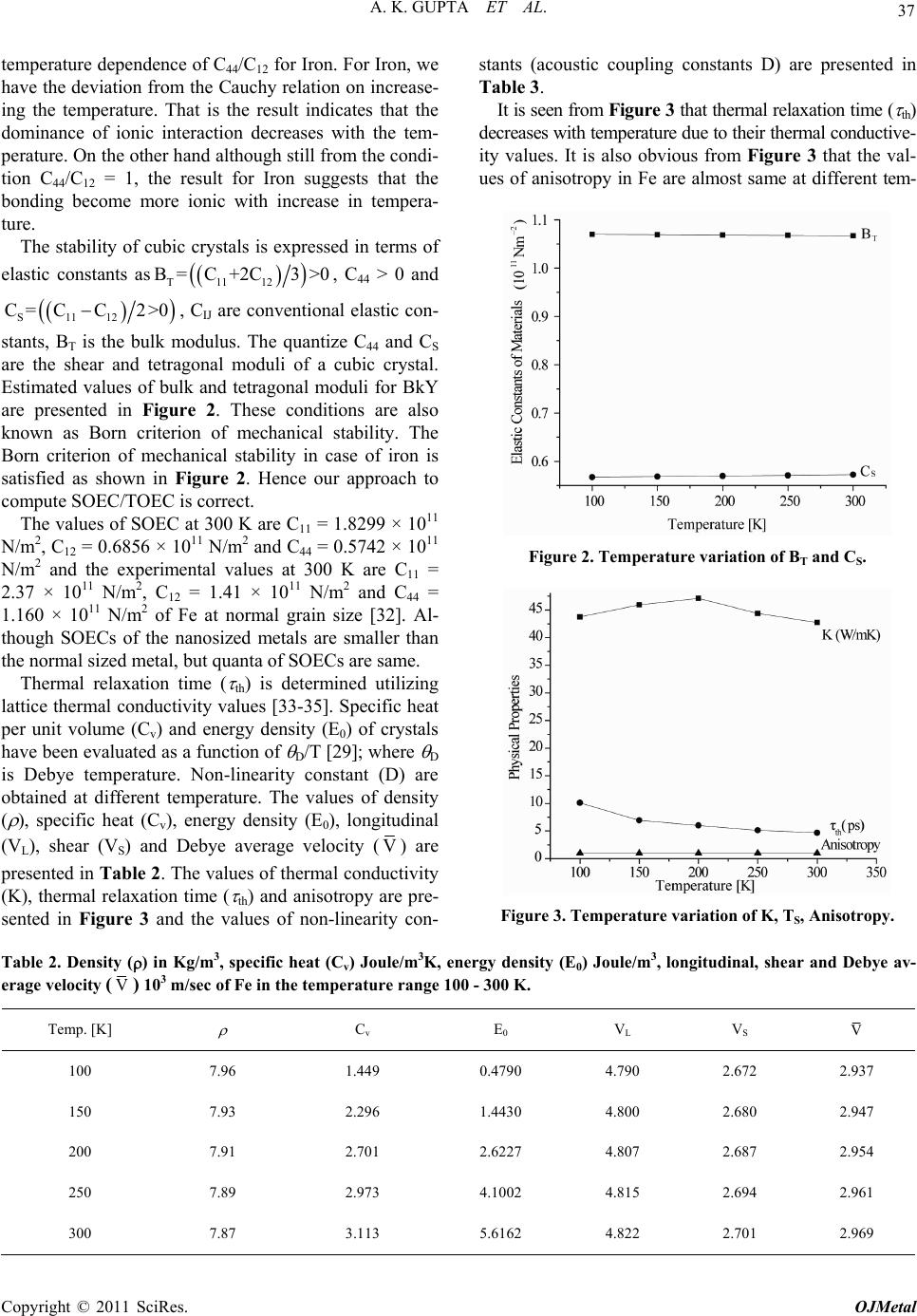 37 A. K. GUPTA ET AL. temperature dependence of C44/C12 for Iron. For Iron, we have the deviation from the Cauchy relation on increase- ing the temperature. That is the result indicates that the dominance of ionic interaction decreases with the tem- perature. On the other hand although still from the condi- tion C44/C12 = 1, the result for Iron suggests that the bonding become more ionic with increase in tempera- ture. The stability of cubic crystals is expressed in terms of elastic constants as T1112 B=C+2C3>0 , C44 > 0 and S1112 C= CC2>0 , CIJ are conventional elastic con- stants, BT is the bulk modulus. The quantize C44 and CS are the shear and tetragonal moduli of a cubic crystal. Estimated values of bulk and tetragonal moduli for BkY are presented in Figure 2. These conditions are also known as Born criterion of mechanical stability. The Born criterion of mechanical stability in case of iron is satisfied as shown in Figure 2. Hence our approach to compute SOEC/TOEC is correct. The values of SOEC at 300 K are C11 = 1.8299 × 1011 N/m2, C12 = 0.6856 × 1011 N/m2 and C44 = 0.5742 × 1011 N/m2 and the experimental values at 300 K are C11 = 2.37 × 1011 N/m2, C12 = 1.41 × 1011 N/m2 and C44 = 1.160 × 1011 N/m2 of Fe at normal grain size [32]. Al- though SOECs of the nanosized metals are smaller than the normal sized metal, but quanta of SOECs are same. Thermal relaxation time ( th) is determined utilizing lattice thermal conductivity values [33-35]. Specific heat per unit volume (Cv) and energy density (E0) of crystals have been evaluated as a function of D/T [29]; where D is Debye temperature. Non-linearity constant (D) are obtained at different temperature. The values of density ( ), specific heat (Cv), energy density (E0), longitudinal (VL), shear (VS) and Debye average velocity (V) are presented in Tabl e 2. The values of thermal conductivity (K), thermal relaxation time ( th) and anisotropy are pre- sented in Figure 3 and the values of non-linearity con- stants (acoustic coupling constants D) are presented in Table 3. It is seen from Figure 3 that thermal relaxation time ( th) decreases with temperature due to their thermal conductive- ity values. It is also obvious from Figure 3 that the val- ues of anisotropy in Fe are almost same at different tem- Figure 2. Temperature variation of BT and CS. Figure 3. Temperature variation of K, TS, Anisotropy. Table 2. Density () in Kg/m3, specific heat (Cv) Joule/m3K, energy density (E0) Joule/m3, longitudinal, shear and Debye av- erage velocity (V) 103 m/sec of Fe in the temperature range 100 - 300 K. Temp. [K] Cv E 0 V L V S V 100 7.96 1.449 0.4790 4.790 2.672 2.937 150 7.93 2.296 1.4430 4.800 2.680 2.947 200 7.91 2.701 2.6227 4.807 2.687 2.954 250 7.89 2.973 4.1002 4.815 2.694 2.961 300 7.87 3.113 5.6162 4.822 2.701 2.969 Copyright © 2011 SciRes. OJMetal
 A. K. GUPTA ET AL. 38 Table 3. Acoustic coupling constants (DL for longitudinal wave, DS for shear wave), along <100>, <110> and <111> crystal- lographic directions of Fe in the temperature range 100 - 300 K. Temperature [K] Direction Parameter 100 150 200 250 300 D L 7.308 7.256 7.186 7.113 7.038 <100> D S 0.648 0.662 0.676 0.691 0.706 D L 12.085 12.096 12.017 11.914 11.790 DS* DS** 0.749 0.769 0.790 0.811 0.833 <110> 12.142 11.964 11.788 11.614 11.442 DL 14.405 14.370 14.041 13.627 13.169 <111> D S*** 15.459 15.133 14.804 14.485 14.178 *Shear wave polarized along <001> direction, **Shear wave polarized alongdirection, ***Shear wave polarized along11 0direction. 110 peratures. The ultrasonic attenuation coefficients (α/f2)Akh. due to p-p interaction and (α/f2)th. due to thermoelastic relaxation in nanosized Fe at 100 - 300 K along <100>, <110> and <111> crystallographic directions are de- termined and the temperature variation of the attenuation is shown in Figures 4-6. A perusal of the Figures 4-6 shows that the thermoelastic loss (α/f2)th. is negligible in comparison to the Akhiezer type attenuation (loss due to p-p interaction). Figure 4. [( /f2)Th(×10–17Nps2·m–1)] vs. Temperature of Fe. Figure 5. [( /f2)Akh.long.(×10–16 Nps2·m–1)] vs. T emperatu re of Fe. Figure 6. [( /f2)Akh.Shear.(×10–16 Nps2·m–1)] vs. Temperature of Fe. This is due to low values of thermal conductivity and higher values of Debye average velocities of the wave along all the three propagating directions. There is posi- tive temperature dependence of the ultrasonic attenuation at high temperatures as in other metals [36,37]. This positive temperature dependence of ultrasonic absorption is due to the fact that p-p interaction occurs at high tem- peratures mainly at room temperature. Order of attenua- tion in the nanosized Fe for longitudinal wave and shear wave is the same as in the like metals [38]. Attenuation of longitudinal wave is more than that of shear wave along <100> and <110> direction polarized along <001> direction. A greater value of ultrasonic at- tenuation for longitudinal wave along <100> in com- parison to shear wave attenuation is due to greater value of non-linearity constants (DL). In case of wave propa- gating along <110> direction and polarized along <001>, although DS* is greater than the DL, the values of (α/f2)Akh.long. are greater than the (α/f2)Akh.shear. This be- haviour is due to smaller value of C44 (SOEC) in compare- son to C11 affecting the wave velocity as per their relations as given below longitudinal 11 V=C andshear 44 V=C , here is the density of the material. In the case of the wave propagating along <111> and Copyright © 2011 SciRes. OJMetal
 39 A. K. GUPTA ET AL. shear wave polarized along 11 0 ; shear attenuation (α/f2)Akh.shear*** is greater than the longitudinal wave at- tenuation (α/f2)Akh.long.. This is due to greater value of shear wave non-linearity constants DS*** in comparison to longitudinal wave non-linearity constants DL. Although no experimental values of attenuation in Fe (bcc) are available for the comparison, but order of attenuation is found same as other bcc metals like potassium, which is studied experimentally by Sathish et al. [39]. Since whole computation is based on only two basic parameters i.e. lattice parameter and hardness parameter, one may conclude that the behaviour of temperature de- pendence of ultrasonic absorption and other allied pa- rameters of nanosized Fe is particular one for the mate- rial and supports our theoretical approach. The preliminary results obtained in this work can be used for further experimental investigation with the pulse echo overlap (PEO) technique for ultrasonic measure- ments. The application of this measurement is mainly in non-destructive testing (NDT) of a material with conven- tional analytical techniques such as polarising micros- copy, X-ray diffraction (XRD), surface tension analysis, solid state nuclear magnetic resonance (NMR), scanning electron microscopy (SEM) and transmission electron microscopy (TEM). 4. References [1] P. A. Elmore and M. A. Breazeale, “Dispersion and Fre- quency Dependent Nonlinearity in a Graphite-Epoxy Com- posite,” Ultrasonics, Vol. 41, No. 9, 2004, pp. 709-718. doi:10.1016/j.ultras.2003.11.001 [2] D. M. Profunser, J. Vollmann and J. Dual, “Determina- tion of the Material Properties of Microstructures by La- ser Based Ultrasound,” Ultrasonics, Vol. 42, No. 1-9, 2004, pp. 641-646. [3] H. Ogi, A. Tsujimoto, S. Nishimura and M. Hirao, “Aco- ustic study of Kinetics of Vacancy Diffusion toward Dis- locations in Aluminum,” Acta Materialia, Vol. 53, No. 2, 2005, pp. 513-517. doi:10.1016/j.actamat.2004.10.007 [4] R. P. Singh and G. S. Verma, “Phonon Conductivity and Acoustic Attenuation in Si,” Physical Review, Vol. 171, No. 3, 1968, pp. 838-842. doi:10.1103/PhysRev.171.838 [5] K. J. Singh, Y. Matsuda, K. Hattori, H. Nakamo and S. Na- gai, “Non-Contact Sound Velocities and Attenuation Mea- surements of Several Ceramics at Elevated Temperatures”, Ultrasonics, Vol. 41, No. 1, 2003, pp. 9-14. doi:10.1016/S0041-624X(02)00392-X [6] R. Esquivel-Sirvent, B. Tan, I., Abdelraziq, S. S. Yun and F. B. Stumpf, “Absorption and Velocity of Ultrasound in Binary Solutions of Poly (Ethylene Glycol) and Water,” Journal of the Acoustical Society of America, Vol. 93, No.2, 1993, pp. 819-820. doi:10.1121/1.405444 [7] S. K. Kor and S. C. Deorani, “Correlation between Acous- tic and Dielectric Relaxation Times in Liquids,” Physical Review Letters, Vol. 27, No. 5, 1971, pp. 242-244. doi:10.1103/PhysRevLett.27.24 [8] S. K. Kor and S. K. Pandey, “Ultrasonic Investigation of Cholesteric Liquid Crystals,” Journal of Chemical Phys- ics, Vol. 64, No. 4, 1976, pp. 1333-1336. doi:10.1103/PhysRevLett.27.24 [9] S. K. Kor, R. R. Yadav and D. Singh, “Ultrasonic Studies of CTAB in Glycol,” Molecular Crystals Liquid Crystals, Vol. 392, No. 1, 2002, pp. 75-81. doi:10.1080.15421400390193918 [10] W. P. Mason, “Piezielectric Crystals and Their Applica- tion to Ultrasonics,” D. Van Nostrand Co. Inc. Princeton, New Jersey, 1950. [11] W. P. Mason, “Physical Acoustics,” Vol. IIIB, Academic Press, New York, 1965. [12] D. Singh, P. K. Yadawa and S. K. Sahu, “Effect of Elec- trical Resistivity on Ultrasonic Attenuation in NpTe,” Cryogenics, Vol. 50, No. 8, 2010, pp. 476-479. doi:10.1103/PhysRevLett.27.24 [13] D. Singh, D. K. Pandey, P. K. Yadawa and A. K. Yadav, “Attenuation of Ultrasonic Waves in V, Nb and Ta at Low Temperature,” Cryogenics, Vol. 49, No. 1, 2009, pp. 12- 16. doi:10.1016/j.cryogenics.2008.08.008 [14] D. Singh, D. K. Pandey, D. K. Pandey and R. R. Yadav, “Propagation of Ultrasonic waves in Neptunium Mono- chalcogenides,” Applied Acoustics, Vol. 72, No. 10, 2011, pp. 737-741. doi:10.1016/j.apacoust.2011.04.002 [15] D. Singh and D. K. Pandey, “Ultrasonic Investigations in Intermetallics,” Pramana-Journal of Physics, Vol. 72, No. 2, 2009, pp. 389-398. doi:10.1007/s12043-009-0034-7 [16] K. Brugger, “Thermodynamic Definition of Higher Order Elastic Coefficients,” Physical Review, Vol. 133, 1964, pp. A1611-A1612. doi:10.1103/PhysRev.133.A1611 [17] M. Born and J. E. Mayer, “Zur Gittertheorie der Lonenk- ristalle,” Zeitschrift für Physik, Vol. 75, No. 1-2, 1932, pp. 1-18. doi:10.1007/BF01340511 [18] P. B. Ghate, “Third-Order Elastic Constants of Alkali Ha- lide Crystals,” Physical Review, Vol. 139, No. 5A, 1965, pp. 1666-1674. doi:10.1103/PhysRev.139.A1666 [19] S. Mori Y. Hiki, “Calculation of the Third- and Fourth- Order Elastic Constants of Alkali Halide Crystals,” Jour- nal of the Physical Society of Japan, Vol. 45, No. 5, 1975, pp. 1449-1456. doi:10.1143/JPSJ.45.1449 [20] R. P. Singh and R. K. Singh, “Theoretical Study of Tem- perature Dependent Lattice Anharmonicity in TlCl and TlBr,” Current Applied Physics, Vol. 10, No. 4, 2010, pp. 1053-1058. doi:10.1016/j.cap.2009.12.040 [21] R. P. Singh and R. K. Singh, “Temperature Dependent Physiccal Effects of Ultrasonic Waves in Beryllium Chalco- genides,” Applied Acoustics, Vol. 71, No. 11, 2010, pp. 328-334. doi:10.1016/j.apacoust.2009.10.005 [22] J. Kumar, Kailash, V. Kumar and A. K. Choudhary, “Tem- perature Dependent of Higher Order Elastic Constants of TeO Crystal,” Asian Journal of Chemistry, Vol. 23, No. 12, 2011, pp. 5601-5604. [23] J. Kumar, Kailash, S. K. Shrivastava, D. Singh and V. Ku- Copyright © 2011 SciRes. OJMetal
 A. K. GUPTA ET AL. Copyright © 2011 SciRes. OJMetal 40 mar, “Ultrasonic Attenuation in Calcium Oxide,” Advances in Materials Physics and Chemistry, Vol. 1, No. 2, 2011, pp. 44-49. doi:/10.4236/ampc.2011.12008 [24] A. Akhiezer, “On the Absorption of Sound in Solids,” Jour- nal of Physics (Moscow), Vol. 1, No.1, 1939, pp. 277-287. [25] H. E. Bömmel and K. Dransfeld, “Excitation and Atte- nuation of Hypersonic Waves in Quartz,” Physical Re- view, Vol. 117, No. 5, 1960, pp. 1245-1252. doi:10.1103/PhysRev.117.1245 [26] T. O. Woodruff and H. Ehrenreich, “Absorption of Sound in Insulators,” Physical Review, Vol. 123, No. 5, 1961, pp. 1553-1559. doi:10.1103/PhysRev.123.1553 [27] W. P. Mason and T. B. Bateman, “Relation between Third- Order Elastic Moduli and the Thermal Attenuation of Ul- trasonic Waves in Nonconducting and Metallic Crystals,” Journal of the Acoustical Society of America, Vol. 40, No. 4, 1966, pp. 852-862. doi:org/10.1121/1.1910158 [28] Y. L. Sun, Y. Dai, L. Q. Zhou and W. Chen, “Single- Crystal Iron Nanowire Arrays,” Solid State Phenomena, Vol. 121-123, No. 3, 2007, pp. 17-20. doi:10.4028/www.sci entifi c.net/SSP.121-123. 17 [29] D. E. Gray, Ed., “AIP Handbook,” 3rd Edition, Mc-Graw Hill Book Co. Inc., New York, 1965. [30] K. Pekala and M. Pekala, “Low Temperature Transport Pro- perties of Nanocrystalline Cu, Fe and Ni,” Nanostruc- tured Materials, Vol. 6, No. 3, 1995, pp. 819-822. doi:10.1016/0965-9773(95)00185-9 [31] R. R. Yadav, D. Singh and A. K. Tiwari, “Ultrasonic Eva- luation in Rare-Earth Metals,” Journal of the Acoustical Society of India, Vol. 30, No. 1-2, 2002, pp. 59-63. [32] L. A. Girifalco and V. G. Weizer, “Application of the Morse Potential Function to Cubic Metals,” Physical Review, Vol. 114, No. 3, 1959, pp. 687-690. doi:10.1103/PhysRev.114.687 [33] Y. S. Touloukian, et al., “Thermal Conductivity: Metallic Elements and Alloys,” TPRS Series, Vol. I, IFI/Plenum, New York, 1970. [34] P. Haen and G. T. Meaden, “The Thermal Conductivity, Thermoelectric Power, and Electrical Resistivity of Tho- rium between 5 and 100°K,” Cryogenics, Vol. 5, No. 4, 1965, pp. 194-198. doi:10.1016/0011-2275(65)90056-1 [35] M. A. Gurry, S. Legvold and F. H. Spedding, “Electrical Re- sistivity of Europium and Ytterbium,” Physical Review B, Vol. 117, No. 4, 1960, pp. 953-954. doi:10.1103/PhysRev.117.953 [36] S. K. Kor, Kailash, K. Shanker and P. Mehrotra, “Behav- iour of Acoustical Phonons in Metals in Low Tempera- ture Region,” Journal of the Physical Society of Japan, Vol. 56, No. 7, 1987, pp. 2428-2432. doi:10.1143/JPSJ.56.2428 [37] S. K. Kor and R. R. Yadav, “Ultrasonic Attenuation Due to Electron-Phonon Interaction in Palladium,” Journal of Pure and Applied Ultrasonics, Vol. 8, No. 3, 1986, pp. 89-90. [38] S. K. Kor and R. K. Singh, “Ultrasonic Attenuation in Al- kali Metals,” Acustica, Vol. 79, No. 2, 1993, pp. 292-295. [39] S. Sathish, S. Chaterjee, O. N. Awasthi and E. S. R. Go- pal, “Electron-Electron Scattering and Ultrasonic Attenu- ation in Potassium,” Journal of Low Temperature Physics, Vol. 63, No. 5-6, 1986, pp. 423-429. doi:10.1007/BF00681490
|