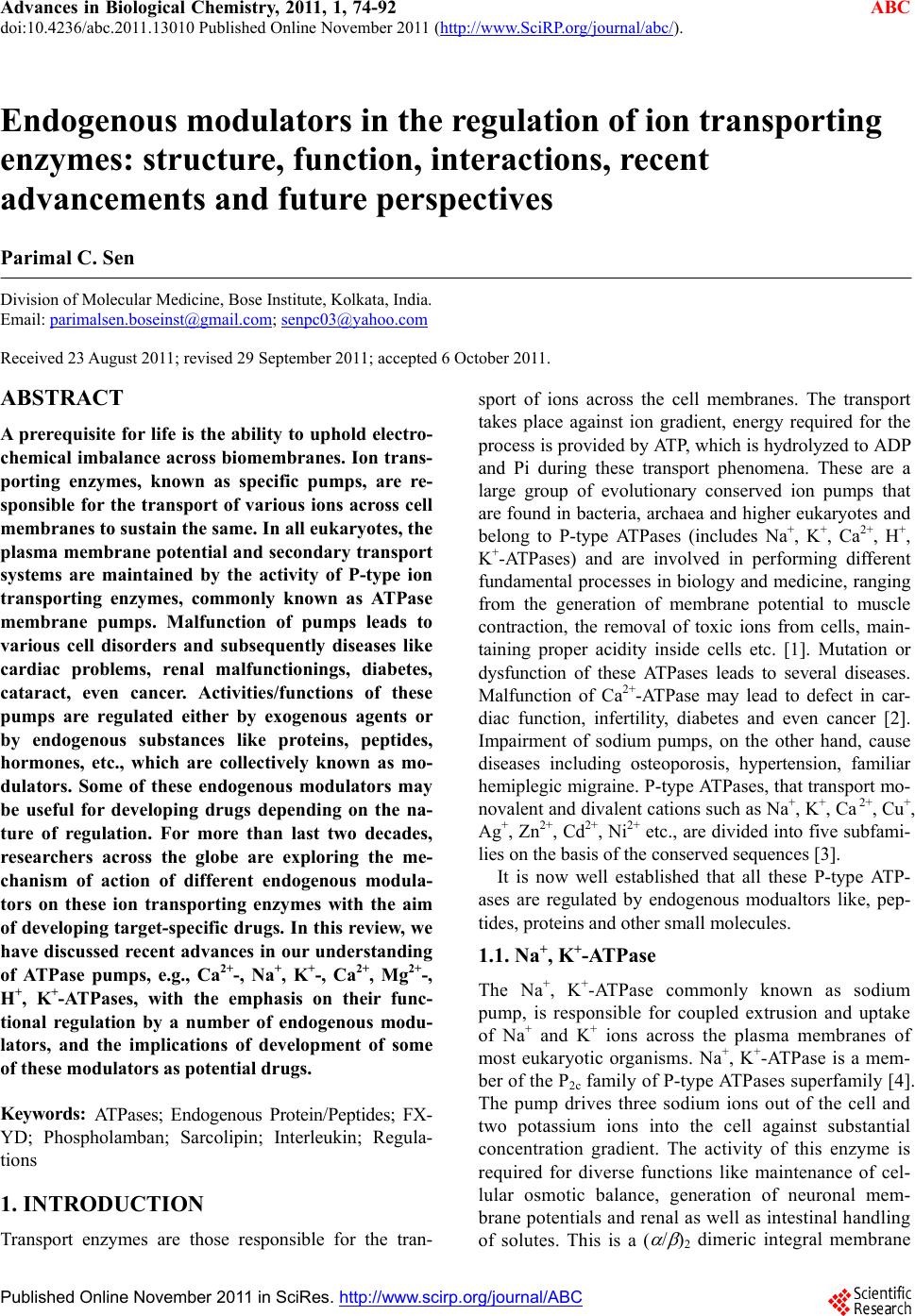 Advances in Biological Chemistry, 2011, 1, 74-92 doi:10.4236/abc.2011.13010 Published Online November 2011 (http://www.SciRP.org/journal/abc/ ABC ). Published Online November 2011 in SciRes. http://www.scirp.org/journal/ABC Endogenous modulators in the regulation of ion transporting enzymes: structure, function, interactions, recent advancements and future perspectives Parimal C. Sen Division of Molecular Medicine, Bose Institute, Kolkata, India. Email: parimalsen.boseinst@gmail.com; senpc03@yahoo.com Received 23 August 2011; revised 29 September 2011; accepted 6 October 2011. ABSTRACT A prerequisite for life is the ability to uphold electro- chemical imbalance across biomembranes. Ion trans- porting enzymes, known as specific pumps, are re- sponsible for the transport of various ions across cell membranes to sustain the same. In all eukaryotes, the plasma membrane potential and secondary transport systems are maintained by the activity of P-type ion transporting enzymes, commonly known as ATPase membrane pumps. Malfunction of pumps leads to various cell disorders and subsequently diseases like cardiac problems, renal malfunctionings, diabetes, cataract, even cancer. Activities/functions of these pumps are regulated either by exogenous agents or by endogenous substances like proteins, peptides, hormones, etc., which are collectively known as mo- dulators. Some of these endogenous modulators may be useful for developing drugs depending on the na- ture of regulation. For more than last two decades, researchers across the globe are exploring the me- chanism of action of different endogenous modula- tors on these ion transporting enzymes with the aim of developing target-specific drugs. In this review, we have discussed recent advances in our understanding of ATPase pumps, e.g., Ca2+-, Na+, K+-, Ca2+, Mg2+-, H+, K+-ATPases, with the emphasis on their func- tional regulation by a number of endogenous modu- lators, and the implications of development of some of these modulators as potential dru gs. Keywords: ATPases; Endogenous Protein/Peptides; FX- YD; Phospholamban; Sarcolipin; Interleukin; Regula- tions 1. INTRODUCTION Transport enzymes are those responsible for the tran- sport of ions across the cell membranes. The transport takes place against ion gradient, energy required for the process is provided by ATP, which is hydrolyzed to ADP and Pi during these transport phenomena. These are a large group of evolutionary conserved ion pumps that are found in bacteria, archaea and higher eukaryotes and belong to P-type ATPases (includes Na+, K+, Ca2+, H+, K+-ATPases) and are involved in performing different fundamental processes in biology and medicine, ranging from the generation of membrane potential to muscle contraction, the removal of toxic ions from cells, main- taining proper acidity inside cells etc. [1]. Mutation or dysfunction of these ATPases leads to several diseases. Malfunction of Ca2+-ATPase may lead to defect in car- diac function, infertility, diabetes and even cancer [2]. Impairment of sodium pumps, on the other hand, cause diseases including osteoporosis, hypertension, familiar hemiplegic migraine. P-type ATPases, that transport mo- novalent and divalent cations such as Na+, K+, Ca 2+, Cu+, Ag+, Zn2+, Cd2+, Ni2+ etc., are divided into five subfami- lies on the basis of the conserved sequences [3]. It is now well established that all these P-type ATP- ases are regulated by endogenous modualtors like, pep- tides, proteins and other small molecules. 1.1. Na+, K+-ATPase The Na+, K+-ATPase commonly known as sodium pump, is responsible for coupled extrusion and uptake of Na+ and K+ ions across the plasma membranes of most eukaryotic organisms. Na+, K+-ATPase is a mem- ber of the P2c family of P-type ATPases superfamily [4]. The pump drives three sodium ions out of the cell and two potassium ions into the cell against substantial concentration gradient. The activity of this enzyme is required for diverse functions like maintenance of cel- lular osmotic balance, generation of neuronal mem- brane potentials and renal as well as intestinal handling of solutes. This is a ( / )2 dimeric integral membrane
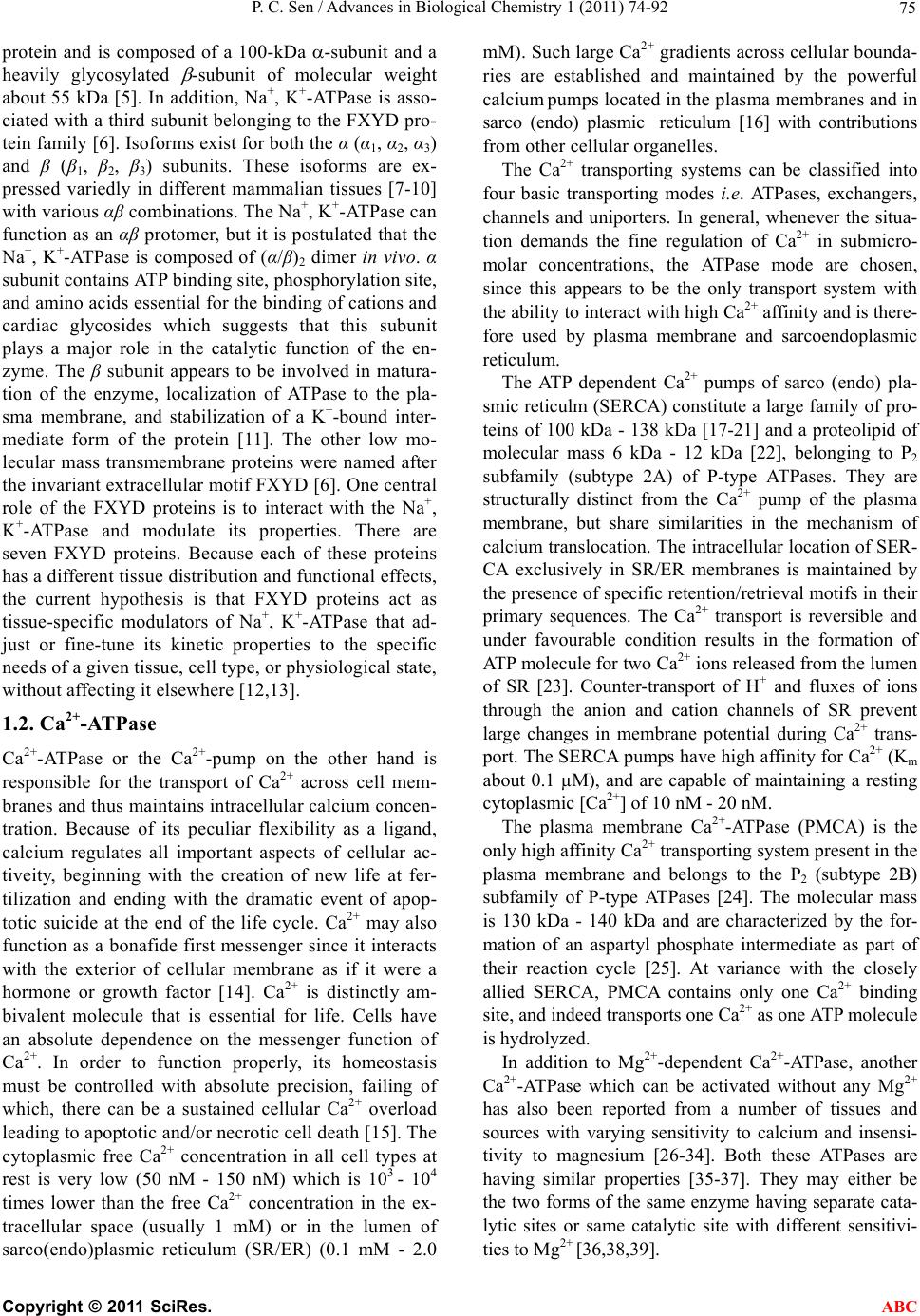 P. C. Sen / Advances in Biological Chemistry 1 (2011) 74-92 75 protein and is composed of a 100-kDa -subunit and a heavily glycosylated -subunit of molecular weight about 55 kDa [5]. In addition, Na+, K+-ATPase is asso- ciated with a third subunit belonging to the FXYD pro- tein family [6]. Isoforms exist for both the α (α1, α2, α3) and β (β1, β2, β3) subunits. These isoforms are ex- pressed variedly in different mammalian tissues [7-10] with various αβ combinations. The Na+, K+-ATPase can function as an αβ protomer, but it is postulated that the Na+, K+-ATPase is composed of (α/β)2 dimer in vivo. α subunit contains ATP binding site, phosphorylation site, and amino acids essential for the binding of cations and cardiac glycosides which suggests that this subunit plays a major role in the catalytic function of the en- zyme. The β subunit appears to be involved in matura- tion of the enzyme, localization of ATPase to the pla- sma membrane, and stabilization of a K+-bound inter- mediate form of the protein [11]. The other low mo- lecular mass transmembrane proteins were named after the invariant extracellular motif FXYD [6]. One central role of the FXYD proteins is to interact with the Na+, K+-ATPase and modulate its properties. There are seven FXYD proteins. Because each of these proteins has a different tissue distribution and functional effects, the current hypothesis is that FXYD proteins act as tissue-specific modulators of Na+, K+-ATPase that ad- just or fine-tune its kinetic properties to the specific needs of a given tissue, cell type, or physiological state, without affecting it elsewhere [12,13]. 1.2. Ca2+-ATPase Ca2+-ATPase or the Ca2+-pump on the other hand is responsible for the transport of Ca2+ across cell mem- branes and thus maintains intracellular calcium concen- tration. Because of its peculiar flexibility as a ligand, calcium regulates all important aspects of cellular ac- tiveity, beginning with the creation of new life at fer- tilization and ending with the dramatic event of apop- totic suicide at the end of the life cycle. Ca2+ may also function as a bonafide first messenger since it interacts with the exterior of cellular membrane as if it were a hormone or growth factor [14]. Ca2+ is distinctly am- bivalent molecule that is essential for life. Cells have an absolute dependence on the messenger function of Ca2+. In order to function properly, its homeostasis must be controlled with absolute precision, failing of which, there can be a sustained cellular Ca2+ overload leading to apoptotic and/or necrotic cell death [15]. The cytoplasmic free Ca2+ concentration in all cell types at rest is very low (50 nM - 150 nM) which is 103 - 104 times lower than the free Ca2+ concentration in the ex- tracellular space (usually 1 mM) or in the lumen of sarco(endo)plasmic reticulum (SR/ER) (0.1 mM - 2.0 mM). Such large Ca2+ gradients across cellular bounda- ries are established and maintained by the powerful calcium pumps located in the plasma membranes and in sarco (endo) plasmic reticulum [16] with contributions from other cellular organelles. The Ca2+ transporting systems can be classified into four basic transporting modes i.e. ATPases, exchangers, channels and uniporters. In general, whenever the situa- tion demands the fine regulation of Ca2+ in submicro- molar concentrations, the ATPase mode are chosen, since this appears to be the only transport system with the ability to interact with high Ca2+ affinity and is there- fore used by plasma membrane and sarcoendoplasmic reticulum. The ATP dependent Ca2+ pumps of sarco (endo) pla- smic reticulm (SERCA) constitute a large family of pro- teins of 100 kDa - 138 kDa [17-21] and a proteolipid of molecular mass 6 kDa - 12 kDa [22], belonging to P2 subfamily (subtype 2A) of P-type ATPases. They are structurally distinct from the Ca2+ pump of the plasma membrane, but share similarities in the mechanism of calcium translocation. The intracellular location of SER- CA exclusively in SR/ER membranes is maintained by the presence of specific retention/retrieval motifs in their primary sequences. The Ca2+ transport is reversible and under favourable condition results in the formation of ATP molecule for two Ca2+ ions released from the lumen of SR [23]. Counter-transport of H+ and fluxes of ions through the anion and cation channels of SR prevent large changes in membrane potential during Ca2+ trans- port. The SERCA pumps have high affinity for Ca2+ (Km about 0.1 µM), and are capable of maintaining a resting cytoplasmic [Ca2+] of 10 nM - 20 nM. The plasma membrane Ca2+-ATPase (PMCA) is the only high affinity Ca2+ transporting system present in the plasma membrane and belongs to the P2 (subtype 2B) subfamily of P-type ATPases [24]. The molecular mass is 130 kDa - 140 kDa and are characterized by the for- mation of an aspartyl phosphate intermediate as part of their reaction cycle [25]. At variance with the closely allied SERCA, PMCA contains only one Ca2+ binding site, and indeed transports one Ca2+ as one ATP molecule is hydrolyzed. In addition to Mg2+-dependent Ca2+-ATPase, another Ca2+-ATPase which can be activated without any Mg2+ has also been reported from a number of tissues and sources with varying sensitivity to calcium and insensi- tivity to magnesium [26-34]. Both these ATPases are having similar properties [35-37]. They may either be the two forms of the same enzyme having separate cata- lytic sites or same catalytic site with different sensitivi- ties to Mg2+ [36,38,39]. C opyright © 2011 SciRes. ABC
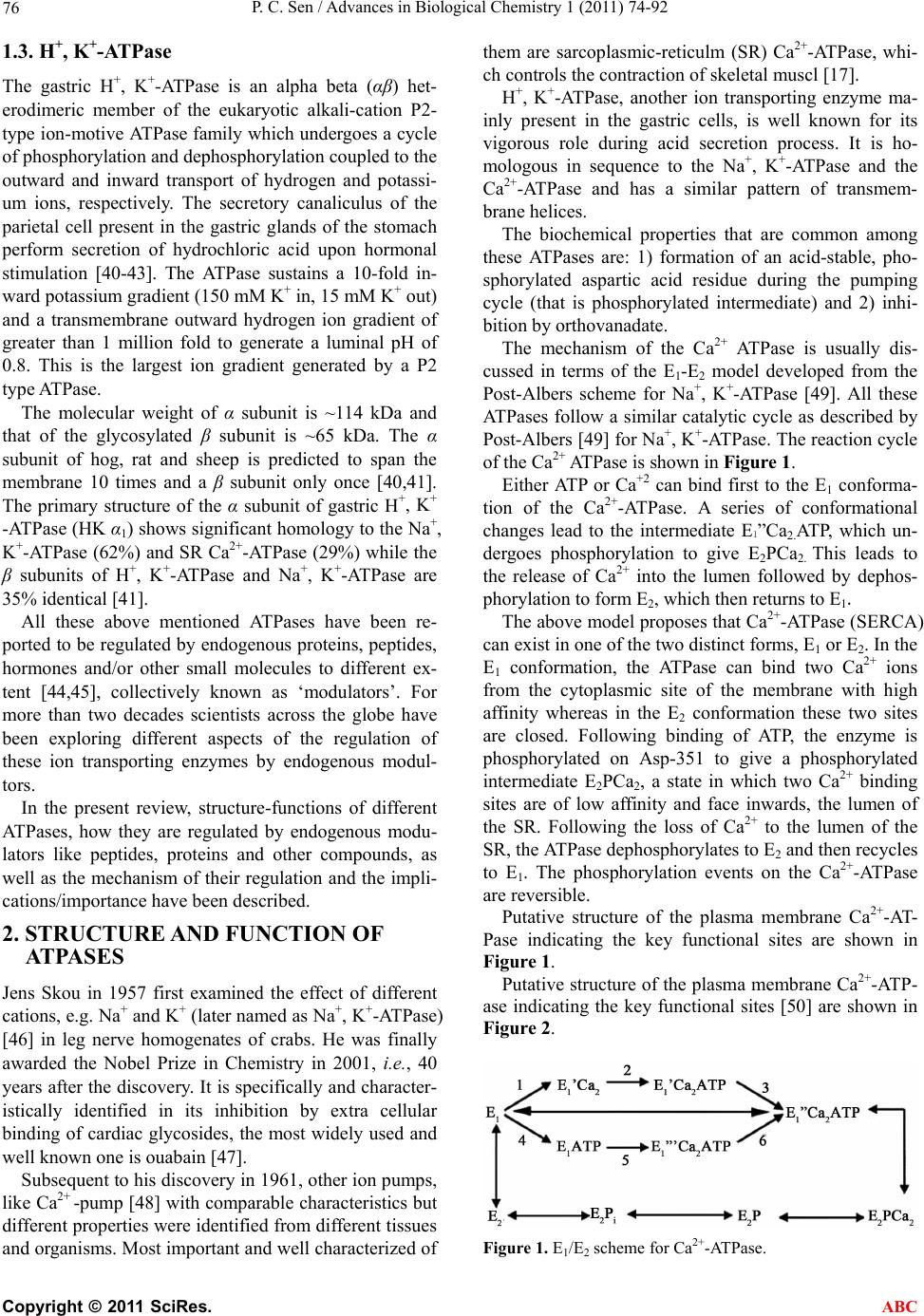 P. C. Sen / Advances in Biological Chemistry 1 (2011) 74-92 Copyright © 2011 SciRes. 76 1.3. H+, K+-ATPase The gastric H+, K+-ATPase is an alpha beta (αβ) het- erodimeric member of the eukaryotic alkali-cation P2- type ion-motive ATPase family which undergoes a cycle of phosphorylation and dephosphorylation coupled to the outward and inward transport of hydrogen and potassi- um ions, respectively. The secretory canaliculus of the parietal cell present in the gastric glands of the stomach perform secretion of hydrochloric acid upon hormonal stimulation [40-43]. The ATPase sustains a 10-fold in- ward potassium gradient (150 mM K+ in, 15 mM K+ out) and a transmembrane outward hydrogen ion gradient of greater than 1 million fold to generate a luminal pH of 0.8. This is the largest ion gradient generated by a P2 type ATPase. ABC The molecular weight of α subunit is ~114 kDa and that of the glycosylated β subunit is ~65 kDa. The α subunit of hog, rat and sheep is predicted to span the membrane 10 times and a β subunit only once [40,41]. The primary structure of the α subunit of gastric H+, K+ -ATPase (HK α1) shows significant homology to the Na+, K+-ATPase (62%) and SR Ca2+-ATPase (29%) while the β subunits of H+, K+-ATPase and Na+, K+-ATPase are 35% identical [41]. All these above mentioned ATPases have been re- ported to be regulated by endogenous proteins, peptides, hormones and/or other small molecules to different ex- tent [44,45], collectively known as ‘modulators’. For more than two decades scientists across the globe have been exploring different aspects of the regulation of these ion transporting enzymes by endogenous modul- tors. In the present review, structure-functions of different ATPases, how they are regulated by endogenous modu- lators like peptides, proteins and other compounds, as well as the mechanism of their regulation and the impli- cations/importance have been described. 2. STRUCTURE AND FUNCTION OF ATPASES Jens Skou in 1957 first examined the effect of different cations, e.g. Na+ and K+ (later named as Na+, K+-ATPase) [46] in leg nerve homogenates of crabs. He was finally awarded the Nobel Prize in Chemistry in 2001, i.e. , 40 years after the discovery. It is specifically and character- istically identified in its inhibition by extra cellular binding of cardiac glycosides, the most widely used and well known one is ouabain [47]. Subsequent to his discovery in 1961, other ion pumps, like Ca2+ -pump [48] with comparable characteristics but different properties were identified from different tissues and organisms. Most important and well characterized of them are sarcoplasmic-reticulm (SR) Ca2+-ATPase, whi- ch controls the contraction of skeletal muscl [17]. H+, K+-ATPase, another ion transporting enzyme ma- inly present in the gastric cells, is well known for its vigorous role during acid secretion process. It is ho- mologous in sequence to the Na+, K+-ATPase and the Ca2+-ATPase and has a similar pattern of transmem- brane helices. The biochemical properties that are common among these ATPases are: 1) formation of an acid-stable, pho- sphorylated aspartic acid residue during the pumping cycle (that is phosphorylated intermediate) and 2) inhi- bition by orthovanadate. The mechanism of the Ca2+ ATPase is usually dis- cussed in terms of the E1-E2 model developed from the Post-Albers scheme for Na+, K+-ATPase [49]. All these ATPases follow a similar catalytic cycle as described by Post-Albers [49] for Na+, K+-ATPase. The reaction cycle of the Ca2+ ATPase is shown in Fi gure 1. Either ATP or Ca+2 can bind first to the E1 conforma- tion of the Ca2+-ATPase. A series of conformational changes lead to the intermediate E1”Ca2.ATP, which un- dergoes phosphorylation to give E2PCa2. This leads to the release of Ca2+ into the lumen followed by dephos- phorylation to form E2, which then returns to E1. The above model proposes that Ca2+-ATPase (SERCA) can exist in one of the two distinct forms, E1 or E2. In the E1 conformation, the ATPase can bind two Ca2+ ions from the cytoplasmic site of the membrane with high affinity whereas in the E2 conformation these two sites are closed. Following binding of ATP, the enzyme is phosphorylated on Asp-351 to give a phosphorylated intermediate E2PCa2, a state in which two Ca2+ binding sites are of low affinity and face inwards, the lumen of the SR. Following the loss of Ca2+ to the lumen of the SR, the ATPase dephosphorylates to E2 and then recycles to E1. The phosphorylation events on the Ca2+-ATPase are reversible. Putative structure of the plasma membrane Ca2+-AT- Pase indicating the key functional sites are shown in Figure 1. Putative structure of the plasma membrane Ca2+-ATP- ase indicating the key functional sites [50] are shown in Figure 2. Figure 1. E1/E2 scheme for Ca2+-ATPase.
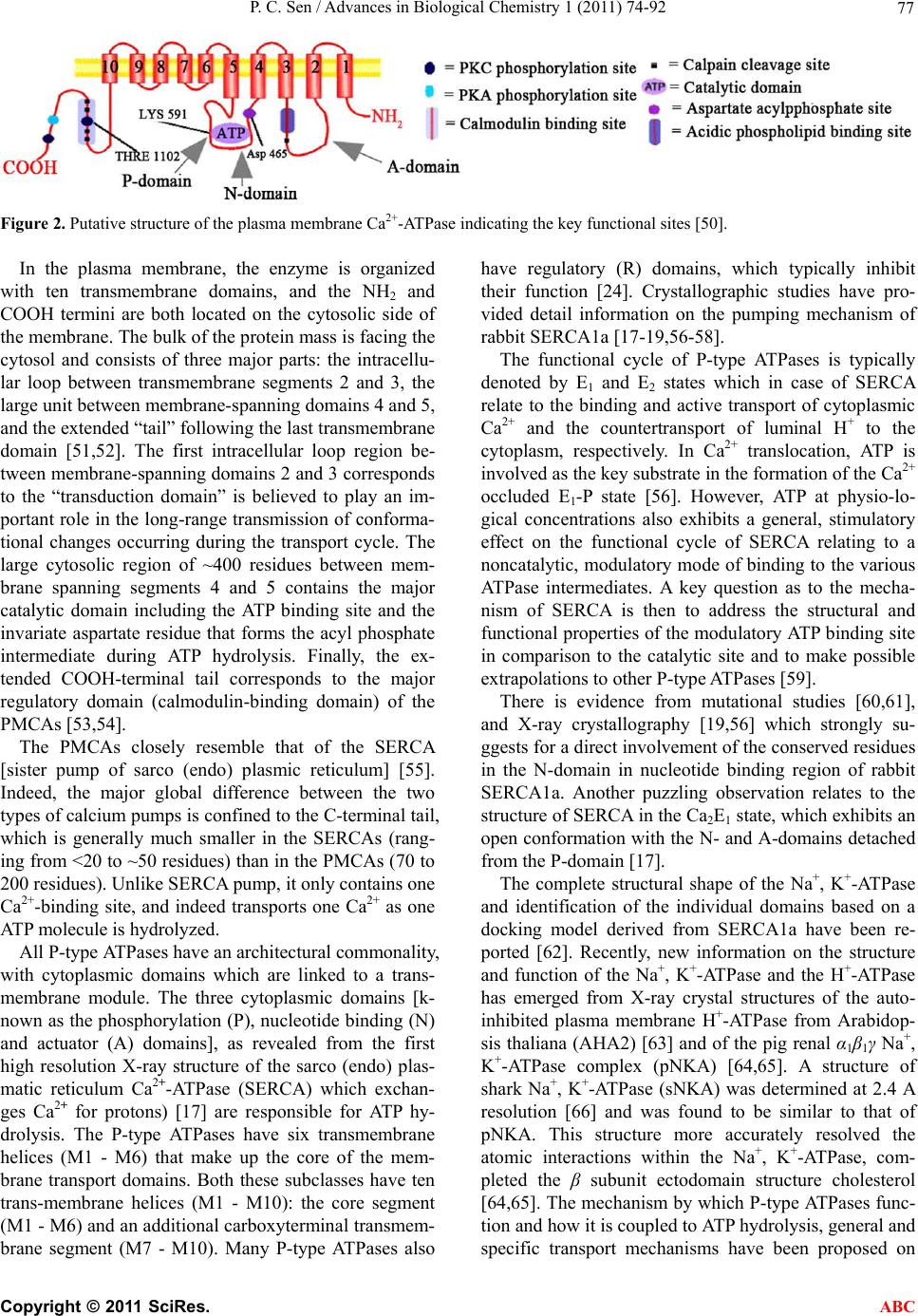 P. C. Sen / Advances in Biological Chemistry 1 (2011) 74-92 77 Figure 2. Putative structure of the plasma membrane Ca2+-ATPase indicating the key functional sites [50]. In the plasma membrane, the enzyme is organized with ten transmembrane domains, and the NH2 and COOH termini are both located on the cytosolic side of the membrane. The bulk of the protein mass is facing the cytosol and consists of three major parts: the intracellu- lar loop between transmembrane segments 2 and 3, the large unit between membrane-spanning domains 4 and 5, and the extended “tail” following the last transmembrane domain [51,52]. The first intracellular loop region be- tween membrane-spanning domains 2 and 3 corresponds to the “transduction domain” is believed to play an im- portant role in the long-range transmission of conforma- tional changes occurring during the transport cycle. The large cytosolic region of ~400 residues between mem- brane spanning segments 4 and 5 contains the major catalytic domain including the ATP binding site and the invariate aspartate residue that forms the acyl phosphate intermediate during ATP hydrolysis. Finally, the ex- tended COOH-terminal tail corresponds to the major regulatory domain (calmodulin-binding domain) of the PMCAs [53,54]. The PMCAs closely resemble that of the SERCA [sister pump of sarco (endo) plasmic reticulum] [55]. Indeed, the major global difference between the two types of calcium pumps is confined to the C-terminal tail, which is generally much smaller in the SERCAs (rang- ing from <20 to ~50 residues) than in the PMCAs (70 to 200 residues). Unlike SERCA pump, it only contains one Ca2+-binding site, and indeed transports one Ca2+ as one ATP molecule is hydrolyzed. All P-type ATPases have an architectural commonality, with cytoplasmic domains which are linked to a trans- membrane module. The three cytoplasmic domains [k- nown as the phosphorylation (P), nucleotide binding (N) and actuator (A) domains], as revealed from the first high resolution X-ray structure of the sarco (endo) plas- matic reticulum Ca2+-ATPase (SERCA) which exchan- ges Ca2+ for protons) [17] are responsible for ATP hy- drolysis. The P-type ATPases have six transmembrane helices (M1 - M6) that make up the core of the mem- brane transport domains. Both these subclasses have ten trans-membrane helices (M1 - M10): the core segment (M1 - M6) and an additional carboxyterminal transmem- brane segment (M7 - M10). Many P-type ATPases also have regulatory (R) domains, which typically inhibit their function [24]. Crystallographic studies have pro- vided detail information on the pumping mechanism of rabbit SERCA1a [17-19,56-58]. The functional cycle of P-type ATPases is typically denoted by E1 and E2 states which in case of SERCA relate to the binding and active transport of cytoplasmic Ca2+ and the countertransport of luminal H+ to the cytoplasm, respectively. In Ca2+ translocation, ATP is involved as the key substrate in the formation of the Ca2+ occluded E1-P state [56]. However, ATP at physio-lo- gical concentrations also exhibits a general, stimulatory effect on the functional cycle of SERCA relating to a noncatalytic, modulatory mode of binding to the various ATPase intermediates. A key question as to the mecha- nism of SERCA is then to address the structural and functional properties of the modulatory ATP binding site in comparison to the catalytic site and to make possible extrapolations to other P-type ATPases [59]. There is evidence from mutational studies [60,61], and X-ray crystallography [19,56] which strongly su- ggests for a direct involvement of the conserved residues in the N-domain in nucleotide binding region of rabbit SERCA1a. Another puzzling observation relates to the structure of SERCA in the Ca2E1 state, which exhibits an open conformation with the N- and A-domains detached from the P-domain [17]. The complete structural shape of the Na+, K+-ATPase and identification of the individual domains based on a docking model derived from SERCA1a have been re- ported [62]. Recently, new information on the structure and function of the Na+, K+-ATPase and the H+-ATPase has emerged from X-ray crystal structures of the auto- inhibited plasma membrane H+-ATPase from Arabidop- sis thaliana (AHA2) [63] and of the pig renal α1β1γ Na+, K+-ATPase complex (pNKA) [64,65]. A structure of shark Na+, K+-ATPase (sNKA) was determined at 2.4 A resolution [66] and was found to be similar to that of pNKA. This structure more accurately resolved the atomic interactions within the Na+, K+-ATPase, com- pleted the β subunit ectodomain structure cholesterol [64,65]. The mechanism by which P-type ATPases func- tion and how it is coupled to ATP hydrolysis, general and specific transport mechanisms have been proposed on C opyright © 2011 SciRes. ABC
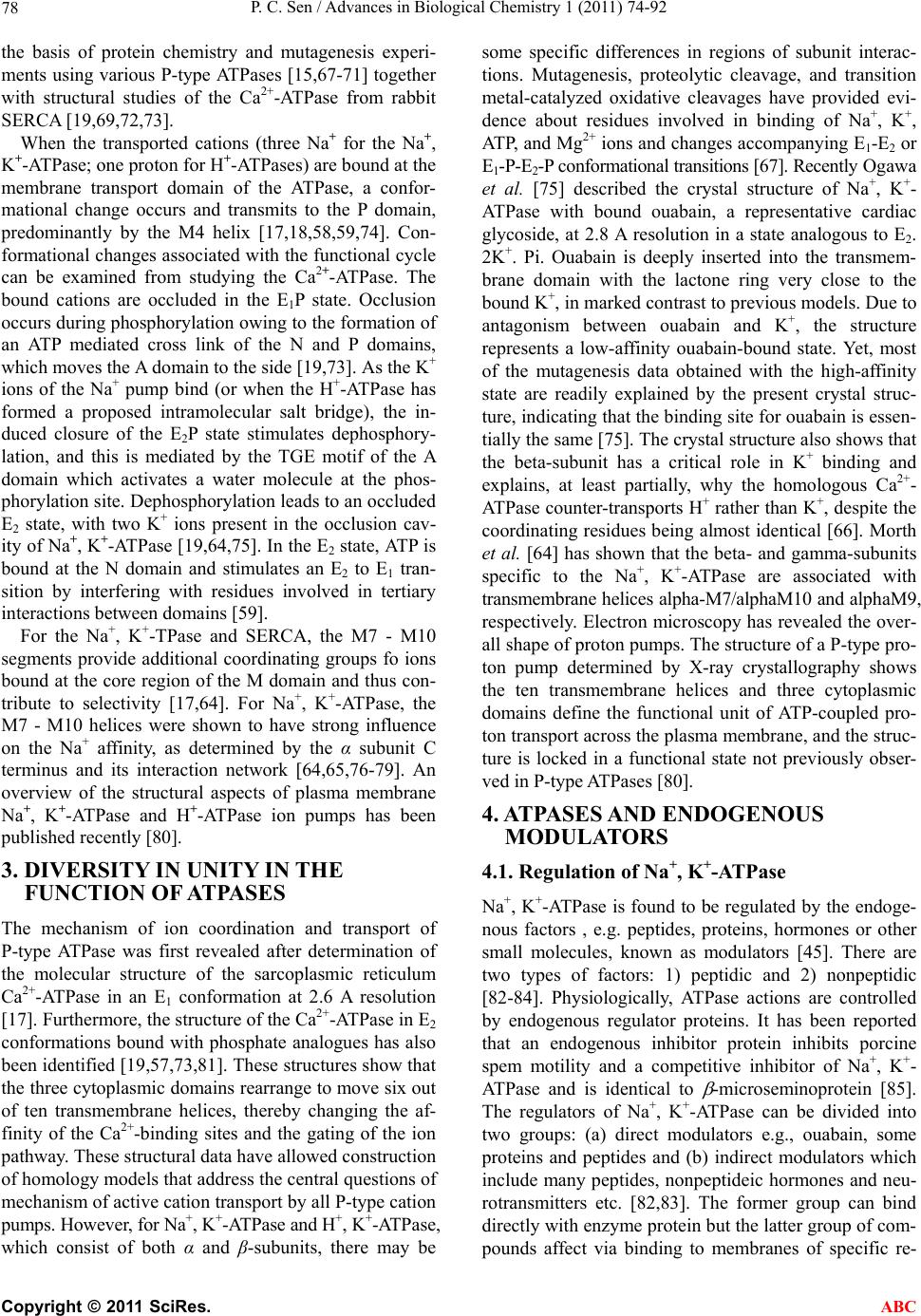 P. C. Sen / Advances in Biological Chemistry 1 (2011) 74-92 78 the basis of protein chemistry and mutagenesis experi- ments using various P-type ATPases [15,67-71] together with structural studies of the Ca2+-ATPase from rabbit SERCA [19,69,72,73]. When the transported cations (three Na+ for the Na+, K+-ATPase; one proton for H+-ATPases) are bound at the membrane transport domain of the ATPase, a confor- mational change occurs and transmits to the P domain, predominantly by the M4 helix [17,18,58,59,74]. Con- formational changes associated with the functional cycle can be examined from studying the Ca2+-ATPase. The bound cations are occluded in the E1P state. Occlusion occurs during phosphorylation owing to the formation of an ATP mediated cross link of the N and P domains, which moves the A domain to the side [19,73]. As the K+ ions of the Na+ pump bind (or when the H+-ATPase has formed a proposed intramolecular salt bridge), the in- duced closure of the E2P state stimulates dephosphory- lation, and this is mediated by the TGE motif of the A domain which activates a water molecule at the phos- phorylation site. Dephosphorylation leads to an occluded E2 state, with two K+ ions present in the occlusion cav- ity of Na+, K+-ATPase [19,64,75]. In the E2 state, ATP is bound at the N domain and stimulates an E2 to E1 tran- sition by interfering with residues involved in tertiary interactions between domains [59]. For the Na+, K+-TPase and SERCA, the M7 - M10 segments provide additional coordinating groups fo ions bound at the core region of the M domain and thus con- tribute to selectivity [17,64]. For Na+, K+-ATPase, the M7 - M10 helices were shown to have strong influence on the Na+ affinity, as determined by the α subunit C terminus and its interaction network [64,65,76-79]. An overview of the structural aspects of plasma membrane Na+, K+-ATPase and H+-ATPase ion pumps has been published recently [80]. 3. DIVERSITY IN UNITY IN THE FUNCTION OF ATPASES The mechanism of ion coordination and transport of P-type ATPase was first revealed after determination of the molecular structure of the sarcoplasmic reticulum Ca2+-ATPase in an E1 conformation at 2.6 A resolution [17]. Furthermore, the structure of the Ca2+-ATPase in E2 conformations bound with phosphate analogues has also been identified [19,57,73,81]. These structures show that the three cytoplasmic domains rearrange to move six out of ten transmembrane helices, thereby changing the af- finity of the Ca2+-binding sites and the gating of the ion pathway. These structural data have allowed construction of homology models that address the central questions of mechanism of active cation transport by all P-type cation pumps. However, for Na+, K+-ATPase and H+, K+-ATPase, which consist of both α and β-subunits, there may be some specific differences in regions of subunit interac- tions. Mutagenesis, proteolytic cleavage, and transition metal-catalyzed oxidative cleavages have provided evi- dence about residues involved in binding of Na+, K+, ATP, and Mg2+ ions and changes accompanying E1-E2 or E1-P-E2-P conformational transitions [67]. Recently Ogawa et al. [75] described the crystal structure of Na+, K+- ATPase with bound ouabain, a representative cardiac glycoside, at 2.8 A resolution in a state analogous to E2. 2K+. Pi. Ouabain is deeply inserted into the transmem- brane domain with the lactone ring very close to the bound K+, in marked contrast to previous models. Due to antagonism between ouabain and K+, the structure represents a low-affinity ouabain-bound state. Yet, most of the mutagenesis data obtained with the high-affinity state are readily explained by the present crystal struc- ture, indicating that the binding site for ouabain is essen- tially the same [75]. The crystal structure also shows that the beta-subunit has a critical role in K+ binding and explains, at least partially, why the homologous Ca2+- ATPase counter-transports H+ rather than K+, despite the coordinating residues being almost identical [66]. Morth et al. [64] has shown that the beta- and gamma-subunits specific to the Na+, K+-ATPase are associated with transmembrane helices alpha-M7/alphaM10 and alphaM9, respectively. Electron microscopy has revealed the over- all shape of proton pumps. The structure of a P-type pro- ton pump determined by X-ray crystallography shows the ten transmembrane helices and three cytoplasmic domains define the functional unit of ATP-coupled pro- ton transport across the plasma membrane, and the struc- ture is locked in a functional state not previously obser- ved in P-type ATPases [80]. 4. ATP ASES AND ENDOGENOUS MODULATORS 4.1. Regulation of Na+, K+-ATPase Na+, K+-ATPase is found to be regulated by the endoge- nous factors , e.g. peptides, proteins, hormones or other small molecules, known as modulators [45]. There are two types of factors: 1) peptidic and 2) nonpeptidic [82-84]. Physiologically, ATPase actions are controlled by endogenous regulator proteins. It has been reported that an endogenous inhibitor protein inhibits porcine spem motility and a competitive inhibitor of Na+, K+- ATPase and is identical to -microseminoprotein [85]. The regulators of Na+, K+-ATPase can be divided into two groups: (a) direct modulators e.g., ouabain, some proteins and peptides and (b) indirect modulators which include many peptides, nonpeptideic hormones and neu- rotransmitters etc. [82,83]. The former group can bind directly with enzyme protein but the latter group of com- pounds affect via binding to membranes of specific re- C opyright © 2011 SciRes. ABC
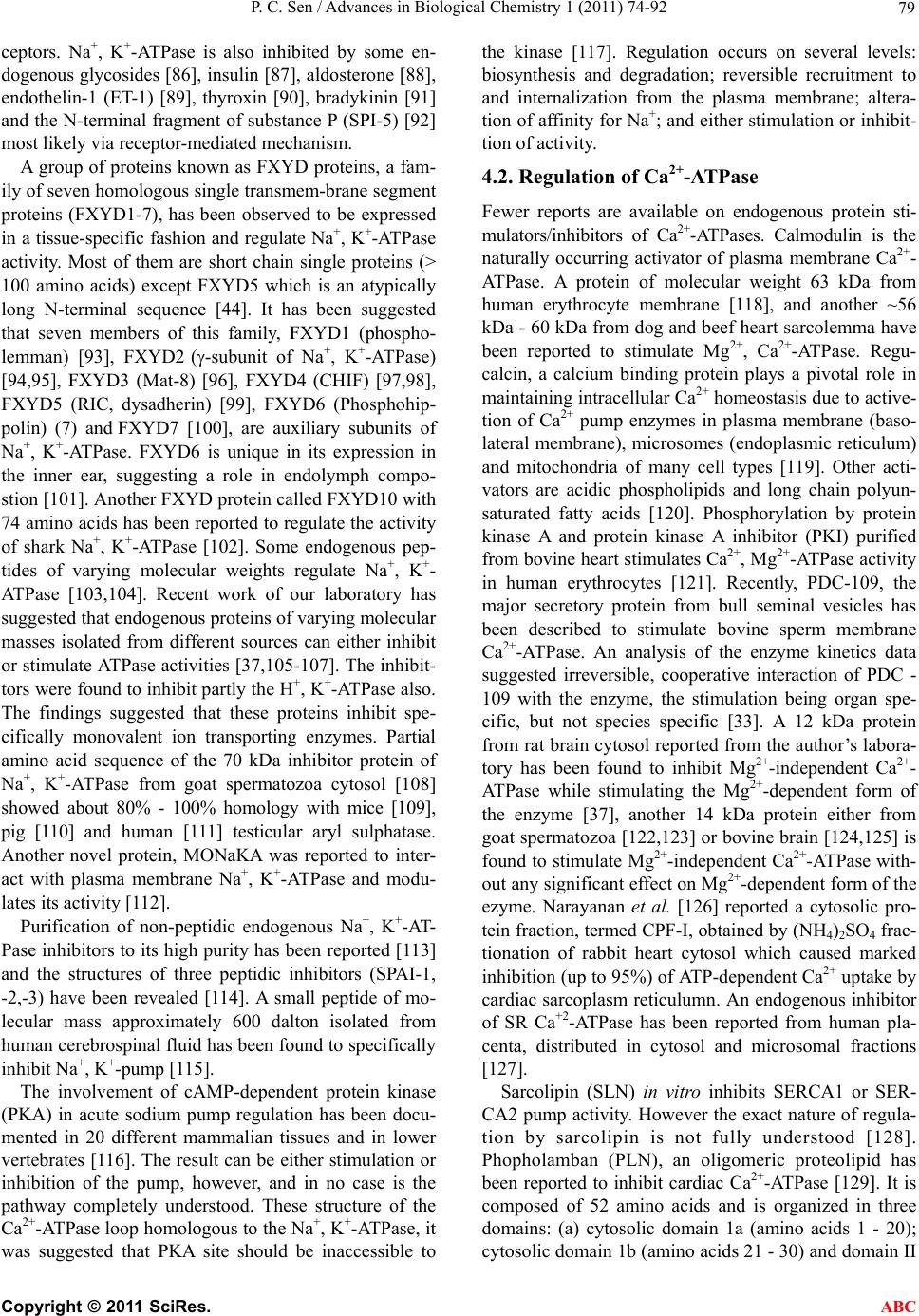 P. C. Sen / Advances in Biological Chemistry 1 (2011) 74-92 79 ceptors. Na+, K+-ATPase is also inhibited by some en- dogenous glycosides [86], insulin [87], aldosterone [88], endothelin-1 (ET-1) [89], thyroxin [90], bradykinin [91] and the N-terminal fragment of substance P (SPI-5) [92] most likely via receptor-mediated mechanism. A group of proteins known as FXYD proteins, a fam- ily of seven homologous single transmem-brane segment proteins (FXYD1-7), has been observed to be expressed in a tissue-specific fashion and regulate Na+, K+-ATPase activity. Most of them are short chain single proteins (> 100 amino acids) except FXYD5 which is an atypically long N-terminal sequence [44]. It has been suggested that seven members of this family, FXYD1 (phospho- lemman) [93], FXYD2 (-subunit of Na+, K+-ATPase) [94,95], FXYD3 (Mat-8) [96], FXYD4 (CHIF) [97,98], FXYD5 (RIC, dysadherin) [99], FXYD6 (Phosphohip- polin) (7) and FXYD7 [100], are auxiliary subunits of Na+, K+-ATPase. FXYD6 is unique in its expression in the inner ear, suggesting a role in endolymph compo- stion [101]. Another FXYD protein called FXYD10 with 74 amino acids has been reported to regulate the activity of shark Na+, K+-ATPase [102]. Some endogenous pep- tides of varying molecular weights regulate Na+, K+- ATPase [103,104]. Recent work of our laboratory has suggested that endogenous proteins of varying molecular masses isolated from different sources can either inhibit or stimulate ATPase activities [37,105-107]. The inhibit- tors were found to inhibit partly the H+, K+-ATPase also. The findings suggested that these proteins inhibit spe- cifically monovalent ion transporting enzymes. Partial amino acid sequence of the 70 kDa inhibitor protein of Na+, K+-ATPase from goat spermatozoa cytosol [108] showed about 80% - 100% homology with mice [109], pig [110] and human [111] testicular aryl sulphatase. Another novel protein, MONaKA was reported to inter- act with plasma membrane Na+, K+-ATPase and modu- lates its activity [112]. Purification of non-peptidic endogenous Na+, K+-AT- Pase inhibitors to its high purity has been reported [113] and the structures of three peptidic inhibitors (SPAI-1, -2,-3) have been revealed [114]. A small peptide of mo- lecular mass approximately 600 dalton isolated from human cerebrospinal fluid has been found to specifically inhibit Na+, K+-pump [115]. The involvement of cAMP-dependent protein kinase (PKA) in acute sodium pump regulation has been docu- mented in 20 different mammalian tissues and in lower vertebrates [116]. The result can be either stimulation or inhibition of the pump, however, and in no case is the pathway completely understood. These structure of the Ca2+-ATPase loop homologous to the Na+, K+-ATPase, it was suggested that PKA site should be inaccessible to the kinase [117]. Regulation occurs on several levels: biosynthesis and degradation; reversible recruitment to and internalization from the plasma membrane; altera- tion of affinity for Na+; and either stimulation or inhibit- tion of activity. 4.2. Regulation of Ca2+-ATPase Fewer reports are available on endogenous protein sti- mulators/inhibitors of Ca2+-ATPases. Calmodulin is the naturally occurring activator of plasma membrane Ca2+- ATPase. A protein of molecular weight 63 kDa from human erythrocyte membrane [118], and another ~56 kDa - 60 kDa from dog and beef heart sarcolemma have been reported to stimulate Mg2+, Ca2+-ATPase. Regu- calcin, a calcium binding protein plays a pivotal role in maintaining intracellular Ca2+ homeostasis due to active- tion of Ca2+ pump enzymes in plasma membrane (baso- lateral membrane), microsomes (endoplasmic reticulum) and mitochondria of many cell types [119]. Other acti- vators are acidic phospholipids and long chain polyun- saturated fatty acids [120]. Phosphorylation by protein kinase A and protein kinase A inhibitor (PKI) purified from bovine heart stimulates Ca2+, Mg2+-ATPase activity in human erythrocytes [121]. Recently, PDC-109, the major secretory protein from bull seminal vesicles has been described to stimulate bovine sperm membrane Ca2+-ATPase. An analysis of the enzyme kinetics data suggested irreversible, cooperative interaction of PDC - 109 with the enzyme, the stimulation being organ spe- cific, but not species specific [33]. A 12 kDa protein from rat brain cytosol reported from the author’s labora- tory has been found to inhibit Mg2+-independent Ca2+- ATPase while stimulating the Mg2+-dependent form of the enzyme [37], another 14 kDa protein either from goat spermatozoa [122,123] or bovine brain [124,125] is found to stimulate Mg2+-independent Ca2+-ATPase with- out any significant effect on Mg2+-dependent form of the ezyme. Narayanan et al. [126] reported a cytosolic pro- tein fraction, termed CPF-I, obtained by (NH4)2SO4 frac- tionation of rabbit heart cytosol which caused marked inhibition (up to 95%) of ATP-dependent Ca2+ uptake by cardiac sarcoplasm reticulumn. An endogenous inhibitor of SR Ca+2-ATPase has been reported from human pla- centa, distributed in cytosol and microsomal fractions [127]. Sarcolipin (SLN) in vitro inhibits SERCA1 or SER- CA2 pump activity. However the exact nature of regula- tion by sarcolipin is not fully understood [128]. Phopholamban (PLN), an oligomeric proteolipid has been reported to inhibit cardiac Ca2+-ATPase [129]. It is composed of 52 amino acids and is organized in three domains: (a) cytosolic domain 1a (amino acids 1 - 20); cytosolic domain 1b (amino acids 21 - 30) and domain II C opyright © 2011 SciRes. ABC
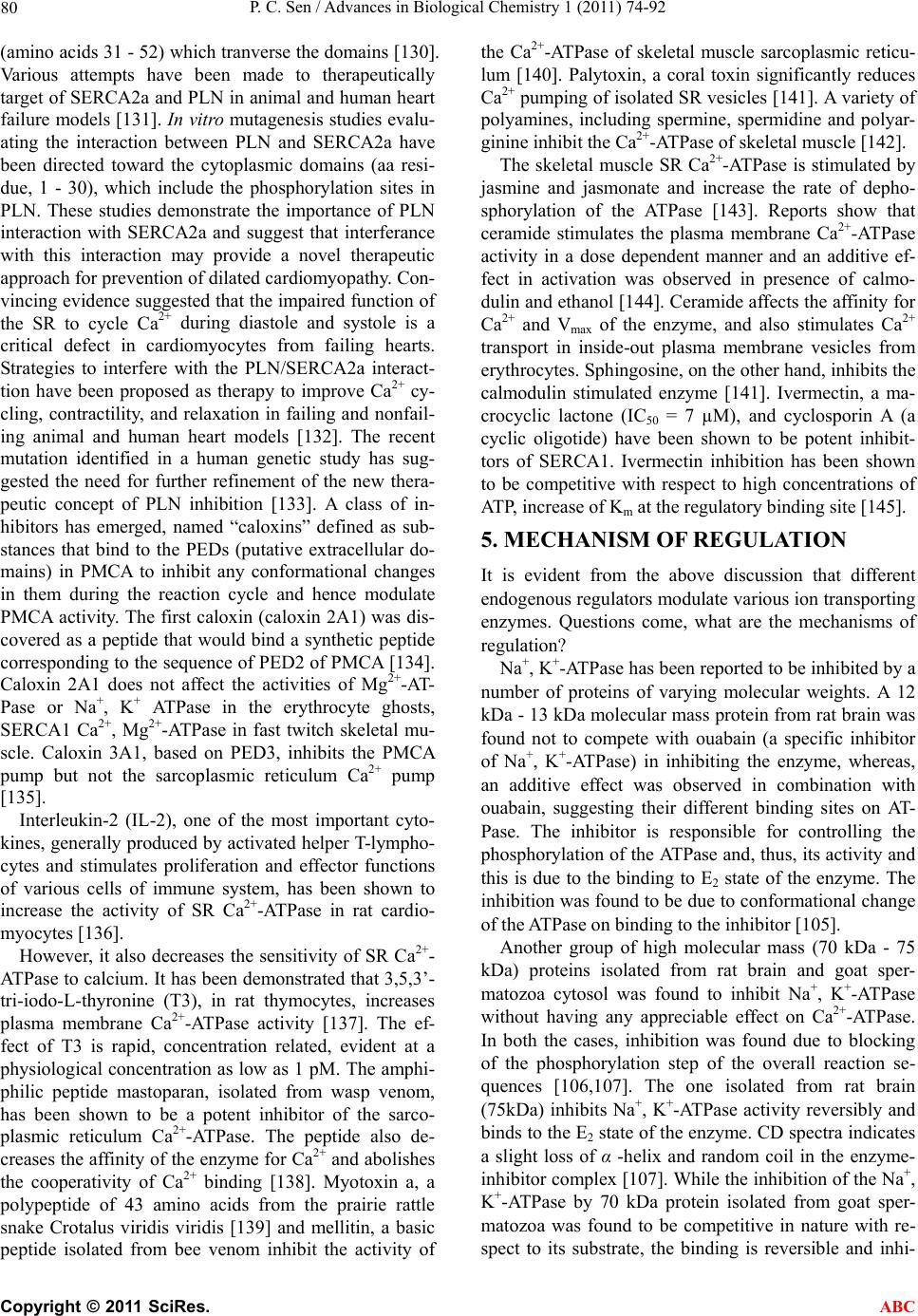 P. C. Sen / Advances in Biological Chemistry 1 (2011) 74-92 80 (amino acids 31 - 52) which tranverse the domains [130]. Various attempts have been made to therapeutically target of SERCA2a and PLN in animal and human heart failure models [131]. In vitro mutagenesis studies evalu- ating the interaction between PLN and SERCA2a have been directed toward the cytoplasmic domains (aa resi- due, 1 - 30), which include the phosphorylation sites in PLN. These studies demonstrate the importance of PLN interaction with SERCA2a and suggest that interferance with this interaction may provide a novel therapeutic approach for prevention of dilated cardiomyopathy. Con- vincing evidence suggested that the impaired function of the SR to cycle Ca2+ during diastole and systole is a critical defect in cardiomyocytes from failing hearts. Strategies to interfere with the PLN/SERCA2a interact- tion have been proposed as therapy to improve Ca2+ cy- cling, contractility, and relaxation in failing and nonfail- ing animal and human heart models [132]. The recent mutation identified in a human genetic study has sug- gested the need for further refinement of the new thera- peutic concept of PLN inhibition [133]. A class of in- hibitors has emerged, named “caloxins” defined as sub- stances that bind to the PEDs (putative extracellular do- mains) in PMCA to inhibit any conformational changes in them during the reaction cycle and hence modulate PMCA activity. The first caloxin (caloxin 2A1) was dis- covered as a peptide that would bind a synthetic peptide corresponding to the sequence of PED2 of PMCA [134]. Caloxin 2A1 does not affect the activities of Mg2+-AT- Pase or Na+, K+ ATPase in the erythrocyte ghosts, SERCA1 Ca2+, Mg2+-ATPase in fast twitch skeletal mu- scle. Caloxin 3A1, based on PED3, inhibits the PMCA pump but not the sarcoplasmic reticulum Ca2+ pump [135]. Interleukin-2 (IL-2), one of the most important cyto- kines, generally produced by activated helper T-lympho- cytes and stimulates proliferation and effector functions of various cells of immune system, has been shown to increase the activity of SR Ca2+-ATPase in rat cardio- myocytes [136]. However, it also decreases the sensitivity of SR Ca2+- ATPase to calcium. It has been demonstrated that 3,5,3’- tri-iodo-L-thyronine (T3), in rat thymocytes, increases plasma membrane Ca2+-ATPase activity [137]. The ef- fect of T3 is rapid, concentration related, evident at a physiological concentration as low as 1 pM. The amphi- philic peptide mastoparan, isolated from wasp venom, has been shown to be a potent inhibitor of the sarco- plasmic reticulum Ca2+-ATPase. The peptide also de- creases the affinity of the enzyme for Ca2+ and abolishes the cooperativity of Ca2+ binding [138]. Myotoxin a, a polypeptide of 43 amino acids from the prairie rattle snake Crotalus viridis viridis [139] and mellitin, a basic peptide isolated from bee venom inhibit the activity of the Ca2+-ATPase of skeletal muscle sarcoplasmic reticu- lum [140]. Palytoxin, a coral toxin significantly reduces Ca2+ pumping of isolated SR vesicles [141]. A variety of polyamines, including spermine, spermidine and polyar- ginine inhibit the Ca2+-ATPase of skeletal muscle [142]. The skeletal muscle SR Ca2+-ATPase is stimulated by jasmine and jasmonate and increase the rate of depho- sphorylation of the ATPase [143]. Reports show that ceramide stimulates the plasma membrane Ca2+-ATPase activity in a dose dependent manner and an additive ef- fect in activation was observed in presence of calmo- dulin and ethanol [144]. Ceramide affects the affinity for Ca2+ and Vmax of the enzyme, and also stimulates Ca2+ transport in inside-out plasma membrane vesicles from erythrocytes. Sphingosine, on the other hand, inhibits the calmodulin stimulated enzyme [141]. Ivermectin, a ma- crocyclic lactone (IC50 = 7 µM), and cyclosporin A (a cyclic oligotide) have been shown to be potent inhibit- tors of SERCA1. Ivermectin inhibition has been shown to be competitive with respect to high concentrations of ATP, increase of Km at the regulatory binding site [145]. 5. MECHANISM OF REGULATION It is evident from the above discussion that different endogenous regulators modulate various ion transporting enzymes. Questions come, what are the mechanisms of regulation? Na+, K+-ATPase has been reported to be inhibited by a number of proteins of varying molecular weights. A 12 kDa - 13 kDa molecular mass protein from rat brain was found not to compete with ouabain (a specific inhibitor of Na+, K+-ATPase) in inhibiting the enzyme, whereas, an additive effect was observed in combination with ouabain, suggesting their different binding sites on AT- Pase. The inhibitor is responsible for controlling the phosphorylation of the ATPase and, thus, its activity and this is due to the binding to E2 state of the enzyme. The inhibition was found to be due to conformational change of the ATPase on binding to the inhibitor [105]. Another group of high molecular mass (70 kDa - 75 kDa) proteins isolated from rat brain and goat sper- matozoa cytosol was found to inhibit Na+, K+-ATPase without having any appreciable effect on Ca2+-ATPase. In both the cases, inhibition was found due to blocking of the phosphorylation step of the overall reaction se- quences [106,107]. The one isolated from rat brain (75kDa) inhibits Na+, K+-ATPase activity reversibly and binds to the E2 state of the enzyme. CD spectra indicates a slight loss of α -helix and random coil in the enzyme- inhibitor complex [107]. While the inhibition of the Na+, K+-ATPase by 70 kDa protein isolated from goat sper- matozoa was found to be competitive in nature with re- spect to its substrate, the binding is reversible and inhi- C opyright © 2011 SciRes. ABC
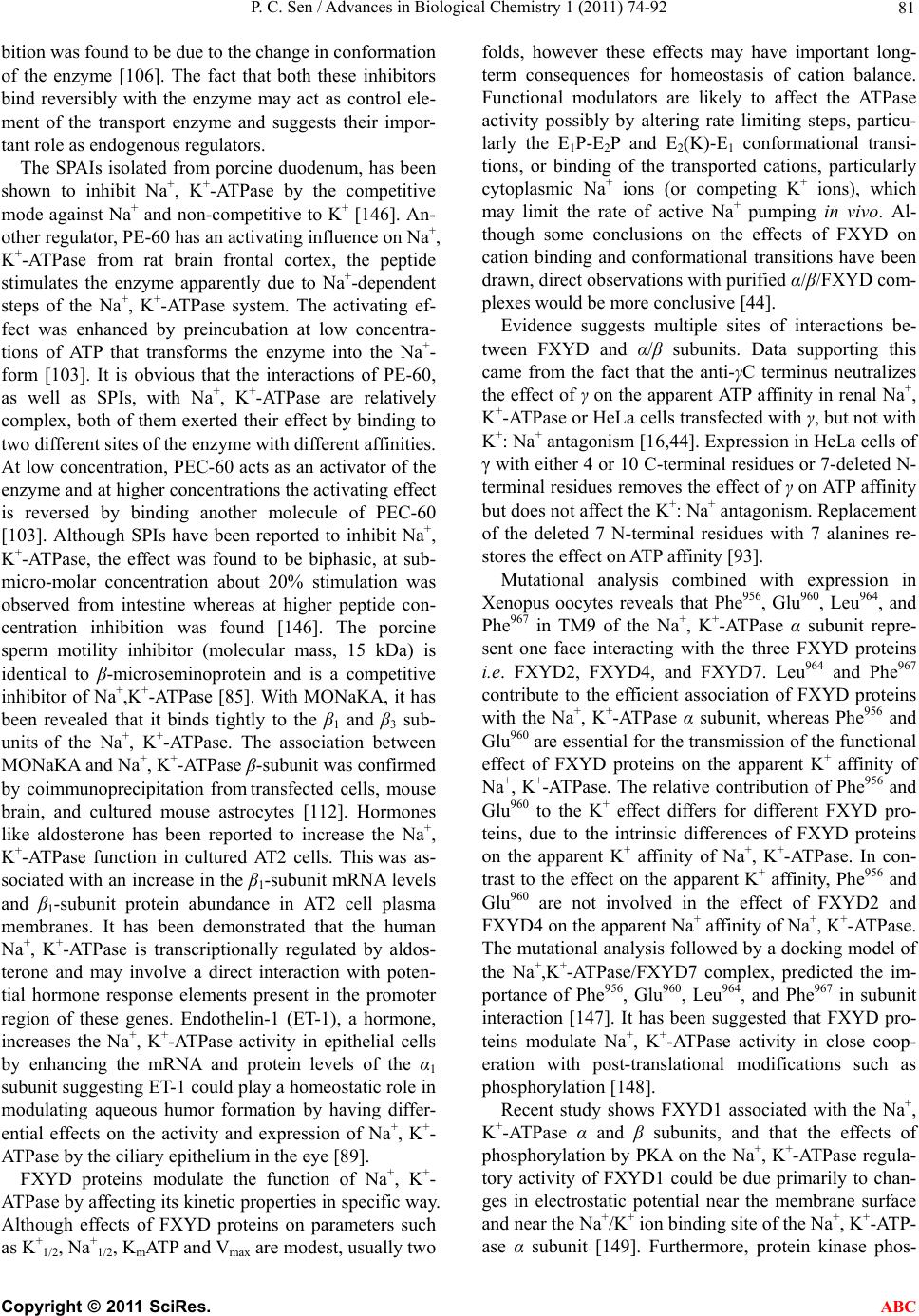 P. C. Sen / Advances in Biological Chemistry 1 (2011) 74-92 81 bition was found to be due to the change in conformation of the enzyme [106]. The fact that both these inhibitors bind reversibly with the enzyme may act as control ele- ment of the transport enzyme and suggests their impor- tant role as endogenous regulators. The SPAIs isolated from porcine duodenum, has been shown to inhibit Na+, K+-ATPase by the competitive mode against Na+ and non-competitive to K+ [146]. An- other regulator, PE-60 has an activating influence on Na+, K+-ATPase from rat brain frontal cortex, the peptide stimulates the enzyme apparently due to Na+-dependent steps of the Na+, K+-ATPase system. The activating ef- fect was enhanced by preincubation at low concentra- tions of ATP that transforms the enzyme into the Na+- form [103]. It is obvious that the interactions of PE-60, as well as SPIs, with Na+, K+-ATPase are relatively complex, both of them exerted their effect by binding to two different sites of the enzyme with different affinities. At low concentration, PEC-60 acts as an activator of the enzyme and at higher concentrations the activating effect is reversed by binding another molecule of PEC-60 [103]. Although SPIs have been reported to inhibit Na+, K+-ATPase, the effect was found to be biphasic, at sub- micro-molar concentration about 20% stimulation was observed from intestine whereas at higher peptide con- centration inhibition was found [146]. The porcine sperm motility inhibitor (molecular mass, 15 kDa) is identical to β-microseminoprotein and is a competitive inhibitor of Na+,K+-ATPase [85]. With MONaKA, it has been revealed that it binds tightly to the β1 and β3 sub- units of the Na+, K+-ATPase. The association between MONaKA and Na+, K+-ATPase β-subunit was confirmed by coimmunoprecipitation from transfected cells, mouse brain, and cultured mouse astrocytes [112]. Hormones like aldosterone has been reported to increase the Na+, K+-ATPase function in cultured AT2 cells. This was as- sociated with an increase in the β1-subunit mRNA levels and β1-subunit protein abundance in AT2 cell plasma membranes. It has been demonstrated that the human Na+, K+-ATPase is transcriptionally regulated by aldos- terone and may involve a direct interaction with poten- tial hormone response elements present in the promoter region of these genes. Endothelin-1 (ET-1), a hormone, increases the Na+, K+-ATPase activity in epithelial cells by enhancing the mRNA and protein levels of the α1 subunit suggesting ET-1 could play a homeostatic role in modulating aqueous humor formation by having differ- ential effects on the activity and expression of Na+, K+- ATPase by the ciliary epithelium in the eye [89]. FXYD proteins modulate the function of Na+, K+- ATPase by affecting its kinetic properties in specific way. Although effects of FXYD proteins on parameters such as K+ 1/2, Na+ 1/2, KmATP and Vmax are modest, usually two folds, however these effects may have important long- term consequences for homeostasis of cation balance. Functional modulators are likely to affect the ATPase activity possibly by altering rate limiting steps, particu- larly the E1P-E2P and E2(K)-E1 conformational transi- tions, or binding of the transported cations, particularly cytoplasmic Na+ ions (or competing K+ ions), which may limit the rate of active Na+ pumping in vivo. Al- though some conclusions on the effects of FXYD on cation binding and conformational transitions have been drawn, direct observations with purified α/β/FXYD com- plexes would be more conclusive [44]. Evidence suggests multiple sites of interactions be- tween FXYD and α/β subunits. Data supporting this came from the fact that the anti-γC terminus neutralizes the effect of γ on the apparent ATP affinity in renal Na+, K+-ATPase or HeLa cells transfected with γ, but not with K+: Na+ antagonism [16,44]. Expression in HeLa cells of γ with either 4 or 10 C-terminal residues or 7-deleted N- terminal residues removes the effect of γ on ATP affinity but does not affect the K+: Na+ antagonism. Replacement of the deleted 7 N-terminal residues with 7 alanines re- stores the effect on ATP affinity [93]. Mutational analysis combined with expression in Xenopus oocytes reveals that Phe956, Glu960, Leu964, and Phe967 in TM9 of the Na+, K+-ATPase α subunit repre- sent one face interacting with the three FXYD proteins i.e. FXYD2, FXYD4, and FXYD7. Leu964 and Phe967 contribute to the efficient association of FXYD proteins with the Na+, K+-ATPase α subunit, whereas Phe956 and Glu960 are essential for the transmission of the functional effect of FXYD proteins on the apparent K+ affinity of Na+, K+-ATPase. The relative contribution of Phe956 and Glu960 to the K+ effect differs for different FXYD pro- teins, due to the intrinsic differences of FXYD proteins on the apparent K+ affinity of Na+, K+-ATPase. In con- trast to the effect on the apparent K+ affinity, Phe956 and Glu960 are not involved in the effect of FXYD2 and FXYD4 on the apparent Na+ affinity of Na+, K+-ATPase. The mutational analysis followed by a docking model of the Na+,K+-ATPase/FXYD7 complex, predicted the im- portance of Phe956, Glu960, Leu964, and Phe967 in subunit interaction [147]. It has been suggested that FXYD pro- teins modulate Na+, K+-ATPase activity in close coop- eration with post-translational modifications such as phosphorylation [148]. Recent study shows FXYD1 associated with the Na+, K+-ATPase α and β subunits, and that the effects of phosphorylation by PKA on the Na+, K+-ATPase regula- tory activity of FXYD1 could be due primarily to chan- ges in electrostatic potential near the membrane surface and near the Na+/K+ ion binding site of the Na+, K+-ATP- ase α subunit [149]. Furthermore, protein kinase phos- C opyright © 2011 SciRes. ABC
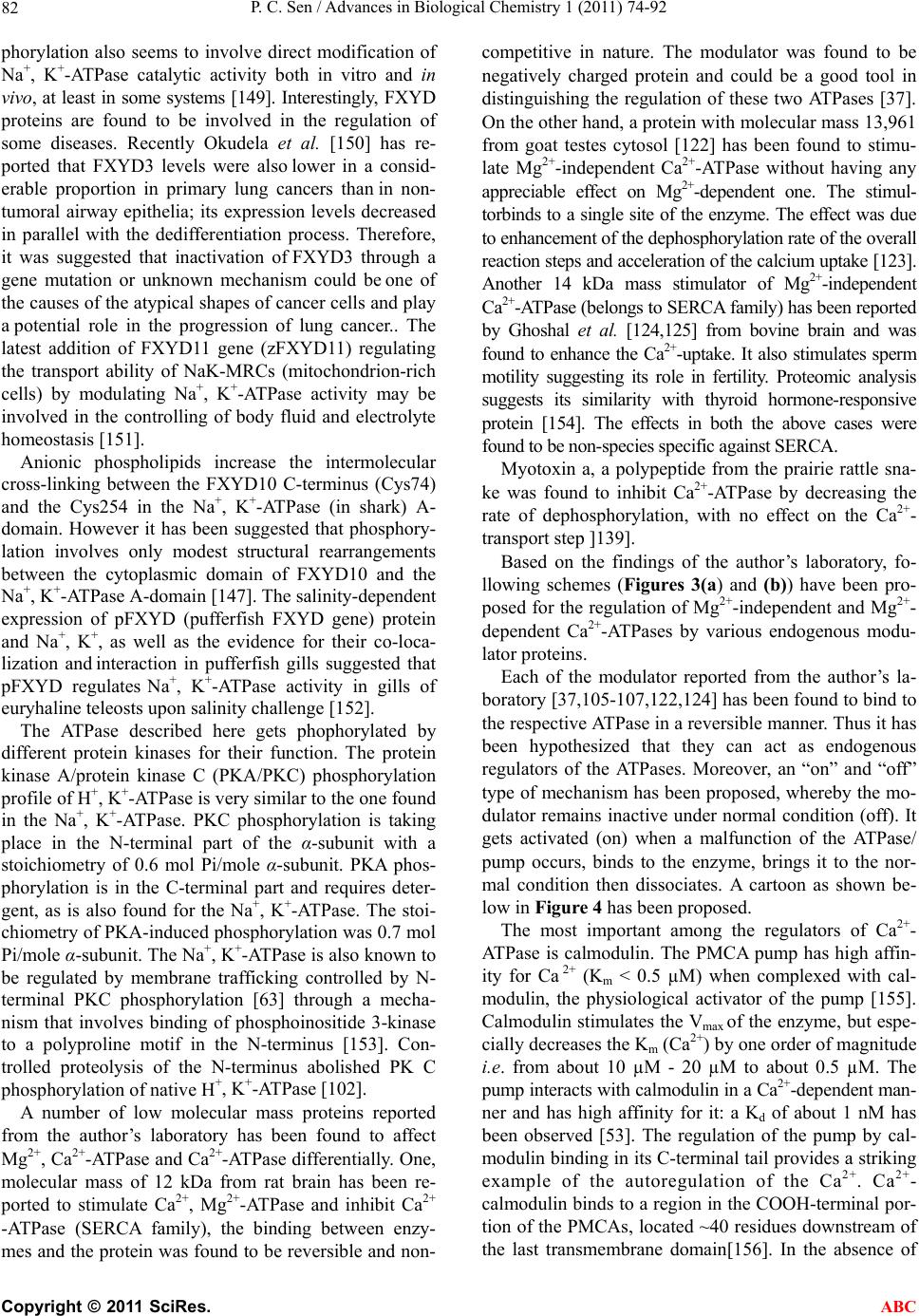 P. C. Sen / Advances in Biological Chemistry 1 (2011) 74-92 Copyright © 2011 SciRes. 82 ABC phorylation also seems to involve direct modification of Na+, K+-ATPase catalytic activity both in vitro and in vivo, at least in some systems [149]. Interestingly, FXYD proteins are found to be involved in the regulation of some diseases. Recently Okudela et al. [150] has re- ported that FXYD3 levels were also lower in a consid- erable proportion in primary lung cancers than in non- tumoral airway epithelia; its expression levels decreased in parallel with the dedifferentiation process. Therefore, it was suggested that inactivation of FXYD3 through a gene mutation or unknown mechanism could be one of the causes of the atypical shapes of cancer cells and play a potential role in the progression of lung cancer.. The latest addition of FXYD11 gene (zFXYD11) regulating the transport ability of NaK-MRCs (mitochondrion-rich cells) by modulating Na+, K+-ATPase activity may be involved in the controlling of body fluid and electrolyte homeostasis [151]. Anionic phospholipids increase the intermolecular cross-linking between the FXYD10 C-terminus (Cys74) and the Cys254 in the Na+, K+-ATPase (in shark) A- domain. However it has been suggested that phosphory- lation involves only modest structural rearrangements between the cytoplasmic domain of FXYD10 and the Na+, K+-ATPase A-domain [147]. The salinity-dependent expression of pFXYD (pufferfish FXYD gene) protein and Na+, K+, as well as the evidence for their co-loca- lization and interaction in pufferfish gills suggested that pFXYD regulates Na+, K+-ATPase activity in gills of euryhaline teleosts upon salinity challenge [152]. The ATPase described here gets phophorylated by different protein kinases for their function. The protein kinase A/protein kinase C (PKA/PKC) phosphorylation profile of H+, K+-ATPase is very similar to the one found in the Na+, K+-ATPase. PKC phosphorylation is taking place in the N-terminal part of the α-subunit with a stoichiometry of 0.6 mol Pi/mole α-subunit. PKA phos- phorylation is in the C-terminal part and requires deter- gent, as is also found for the Na+, K+-ATPase. The stoi- chiometry of PKA-induced phosphorylation was 0.7 mol Pi/mole α-subunit. The Na+, K+-ATPase is also known to be regulated by membrane trafficking controlled by N- terminal PKC phosphorylation [63] through a mecha- nism that involves binding of phosphoinositide 3-kinase to a polyproline motif in the N-terminus [153]. Con- trolled proteolysis of the N-terminus abolished PK C phosphorylation of native H+, K+-ATPase [102]. A number of low molecular mass proteins reported from the author’s laboratory has been found to affect Mg2+, Ca2+-ATPase and Ca2+-ATPase differentially. One, molecular mass of 12 kDa from rat brain has been re- ported to stimulate Ca2+, Mg2+-ATPase and inhibit Ca2+ -ATPase (SERCA family), the binding between enzy- mes and the protein was found to be reversible and non- competitive in nature. The modulator was found to be negatively charged protein and could be a good tool in distinguishing the regulation of these two ATPases [37]. On the other hand, a protein with molecular mass 13,961 from goat testes cytosol [122] has been found to stimu- late Mg2+-independent Ca2+-ATPase without having any appreciable effect on Mg2+-dependent one. The stimul- torbinds to a single site of the enzyme. The effect was due to enhancement of the dephosphorylation rate of the overall reaction steps and acceleration of the calcium uptake [123]. Another 14 kDa mass stimulator of Mg2+-independent Ca2+-ATPase (belongs to SERCA family) has been reported by Ghoshal et al. [124,125] from bovine brain and was found to enhance the Ca2+-uptake. It also stimulates sperm motility suggesting its role in fertility. Proteomic analysis suggests its similarity with thyroid hormone-responsive protein [154]. The effects in both the above cases were found to be non-species specific against SERCA. Myotoxin a, a polypeptide from the prairie rattle sna- ke was found to inhibit Ca2+-ATPase by decreasing the rate of dephosphorylation, with no effect on the Ca2+- transport step ]139]. Based on the findings of the author’s laboratory, fo- llowing schemes (Figures 3(a) and (b)) have been pro- posed for the regulation of Mg2+-independent and Mg2+- dependent Ca2+-ATPases by various endogenous modu- lator proteins. Each of the modulator reported from the author’s la- boratory [37,105-107,122,124] has been found to bind to the respective ATPase in a reversible manner. Thus it has been hypothesized that they can act as endogenous regulators of the ATPases. Moreover, an “on” and “off” type of mechanism has been proposed, whereby the mo- dulator remains inactive under normal condition (off). It gets activated (on) when a malfunction of the ATPase/ pump occurs, binds to the enzyme, brings it to the nor- mal condition then dissociates. A cartoon as shown be- low in Figur e 4 has been proposed. The most important among the regulators of Ca2+- ATPase is calmodulin. The PMCA pump has high affin- ity for Ca 2+ (Km < 0.5 µM) when complexed with cal- modulin, the physiological activator of the pump [155]. Calmodulin stimulates the Vmax of the enzyme, but espe- cially decreases the Km (Ca2+) by one order of magnitude i.e. from about 10 µM - 20 µM to about 0.5 µM. The pump interacts with calmodulin in a Ca2+-dependent man- ner and has high affinity for it: a Kd of about 1 nM has been observed [53]. The regulation of the pump by cal- modulin binding in its C-terminal tail provides a striking example of the autoregulation of the Ca2+. Ca2+- calmodulin binds to a region in the COOH-terminal por- tion of the PMCAs, located ~40 residues downstream of the last transmembrane domain[156]. In the absence of
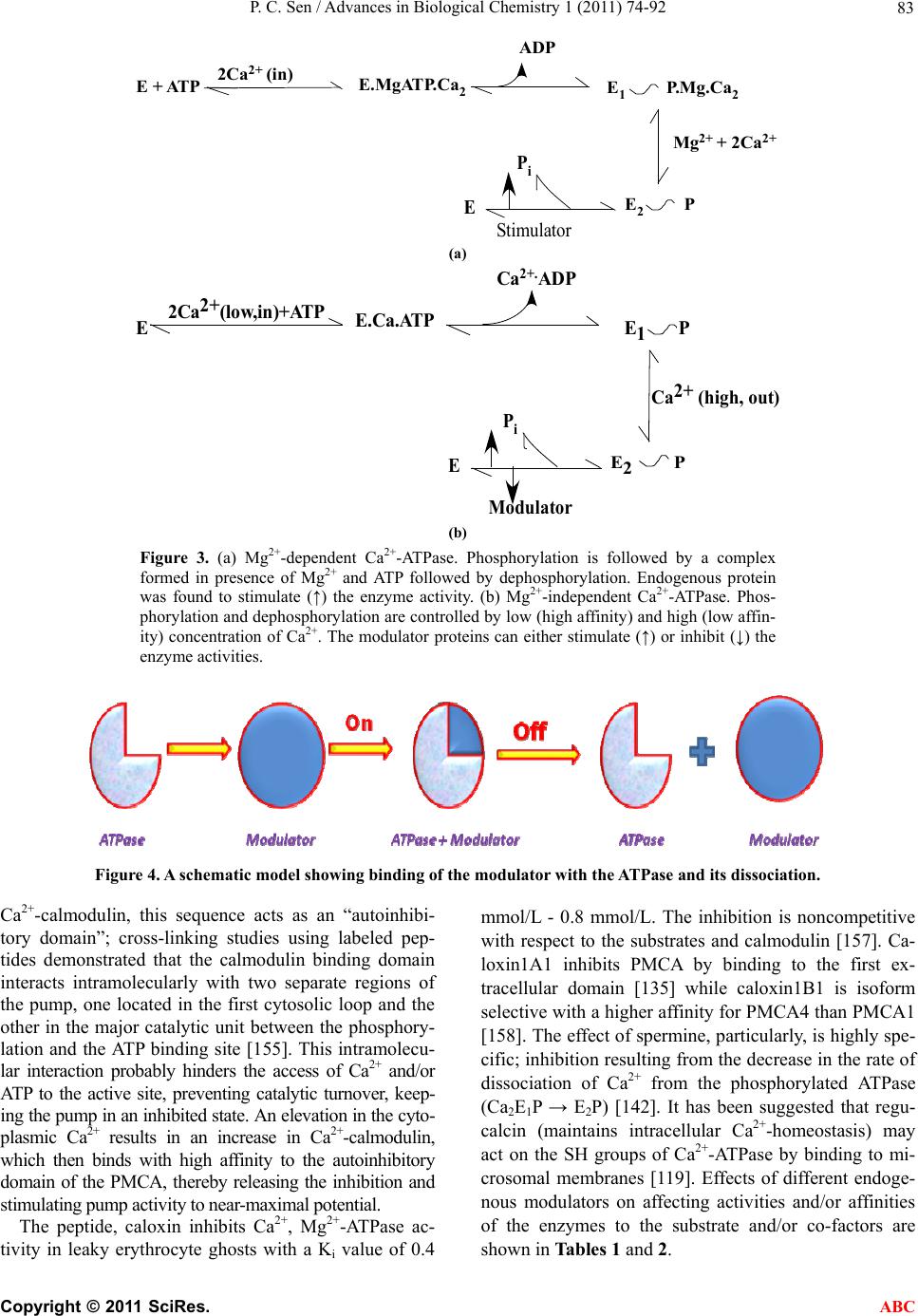 P. C. Sen / Advances in Biological Chemistry 1 (2011) 74-92 83 E+ATP2Ca 2+ (in) E.MgATP.Ca 2 DP E 2 P Mg 2+ +2Ca 2+ P i E S u a o E 1 P.Mg.Ca 2 (a) E2Ca2+(low,in)+ATP E.Ca.AT P a 2 . E1P E2P Ca2+ (high,out) E Mo tor P i (b) Figure 3. (a) Mg2+-dependent Ca2+-ATPase. Phosphorylation is followed by a complex formed in presence of Mg2+ and ATP followed by dephosphorylation. Endogenous protein was found to stimulate (↑) the enzyme activity. (b) Mg2+-independent Ca2+-ATPase. Phos- phorylation and dephosphorylation are controlled by low (high affinity) and high (low affin- ity) concentration of Ca2+. The modulator proteins can either stimulate (↑) or inhibit (↓) the enzyme activities. Figure 4. A sc hematic model showing binding of the modulator with the ATPase and its dissociation. Ca2+-calmodulin, this sequence acts as an “autoinhibi- tory domain”; cross-linking studies using labeled pep- tides demonstrated that the calmodulin binding domain interacts intramolecularly with two separate regions of the pump, one located in the first cytosolic loop and the other in the major catalytic unit between the phosphory- lation and the ATP binding site [155]. This intramolecu- lar interaction probably hinders the access of Ca2+ and/or ATP to the active site, preventing catalytic turnover, keep- ing the pump in an inhibited state. An elevation in the cyto- plasmic Ca2+ results in an increase in Ca2+-calmodulin, which then binds with high affinity to the autoinhibitory domain of the PMCA, thereby releasing the inhibition and stimulating pump activity to near-maximal potential. The peptide, caloxin inhibits Ca2+, Mg2+-ATPase ac- tivity in leaky erythrocyte ghosts with a Ki value of 0.4 mmol/L - 0.8 mmol/L. The inhibition is noncompetitive with respect to the substrates and calmodulin [157]. Ca- loxin1A1 inhibits PMCA by binding to the first ex- tracellular domain [135] while caloxin1B1 is isoform selective with a higher affinity for PMCA4 than PMCA1 [158]. The effect of spermine, particularly, is highly spe- cific; inhibition resulting from the decrease in the rate of dissociation of Ca2+ from the phosphorylated ATPase (Ca2E1P → E2P) [142]. It has been suggested that regu- calcin (maintains intracellular Ca2+-homeostasis) may act on the SH groups of Ca2+-ATPase by binding to mi- crosomal membranes [119]. Effects of different endoge- nous modulators on affecting activities and/or affinities of the enzymes to the substrate and/or co-factors are shown in Tables 1 and 2. C opyright © 2011 SciRes. ABC
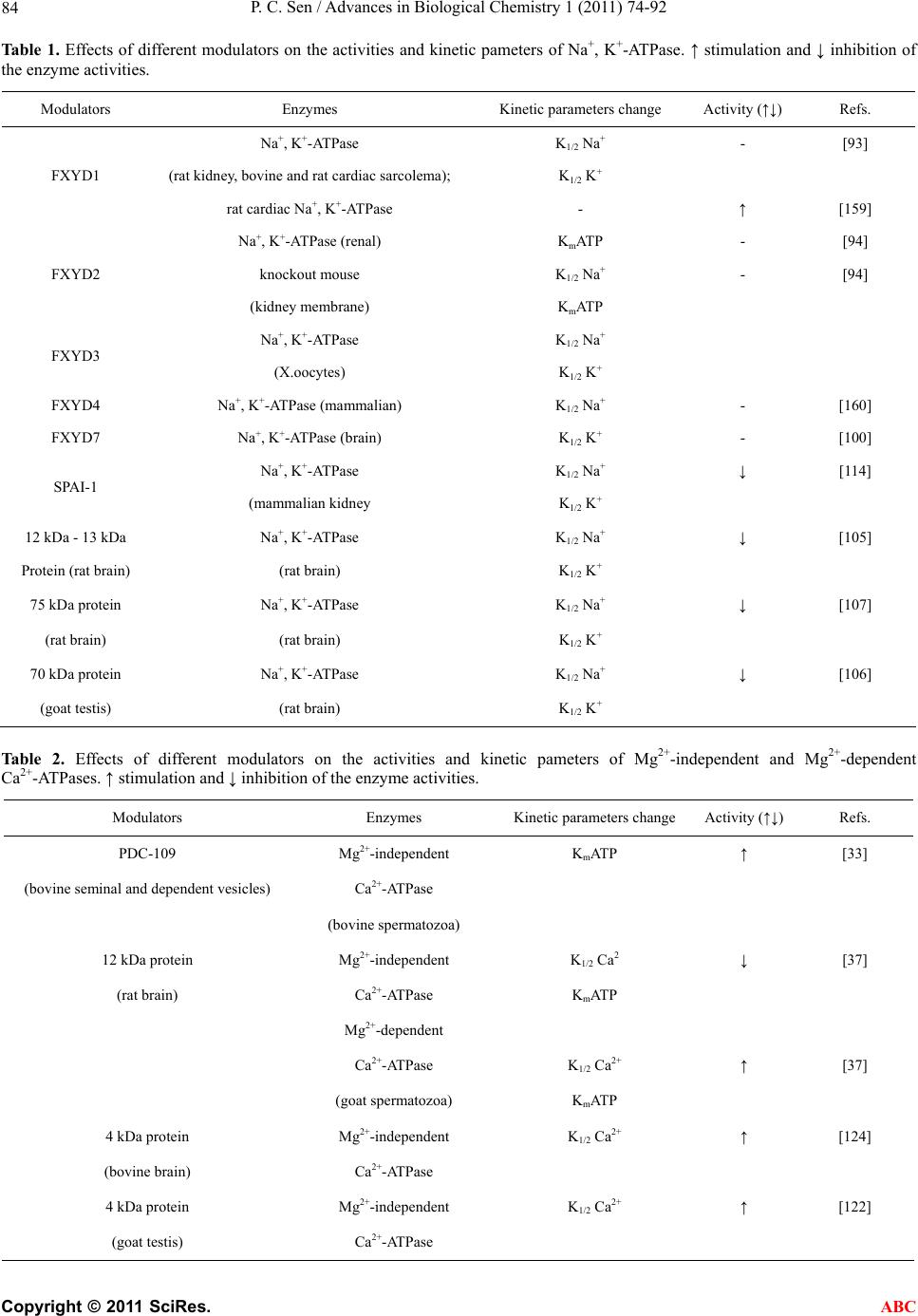 P. C. Sen / Advances in Biological Chemistry 1 (2011) 74-92 84 Ta ble 1. Effects of different modulators on the activities and kinetic pameters of Na+, K+-ATPase. ↑ stimulation and ↓ inhibition of the enzyme activities. Modulators Enzymes Kinetic parameters change Activity (↑↓) Refs. Na+, K+-ATPase K1/2 Na+ - [93] (rat kidney, bovine and rat cardiac sarcolema); K1/2 K+ FXYD1 rat cardiac Na+, K+-ATPase - ↑ [159] Na+, K+-ATPase (renal) KmATP - [94] knockout mouse K1/2 Na+ - [94] FXYD2 (kidney membrane) KmATP Na+, K+-ATPase K1/2 Na+ FXYD3 (X.oocytes) K1/2 K+ FXYD4 Na+, K+-ATPase (mammalian) K1/2 Na+ - [160] FXYD7 Na+, K+-ATPase (brain) K1/2 K+ - [100] Na+, K+-ATPase K1/2 Na+ ↓ [114] SPAI-1 (mammalian kidney K1/2 K+ 12 kDa - 13 kDa Na+, K+-ATPase K1/2 Na+ ↓ [105] Protein (rat brain) (rat brain) K1/2 K+ 75 kDa protein Na+, K+-ATPase K1/2 Na+ ↓ [107] (rat brain) (rat brain) K1/2 K+ 70 kDa protein Na+, K+-ATPase K1/2 Na+ ↓ [106] (goat testis) (rat brain) K1/2 K+ Table 2. Effects of different modulators on the activities and kinetic pameters of Mg2+-independent and Mg2+-dependent Ca2+-ATPases. ↑ stimulation and ↓ inhibition of the enzyme activities. Modulators Enzymes Kinetic parameters change Activity (↑↓) Refs. PDC-109 Mg2+-independent KmAT P ↑ [33] (bovine seminal and dependent vesicles) Ca2+-ATPase (bovine spermatozoa) 12 kDa protein Mg2+-independent K1/2 Ca2 ↓ [37] (rat brain) Ca2+-ATPase KmATP Mg2+-dependent Ca2+-ATPase K1/2 Ca2+ ↑ [37] (goat spermatozoa) KmATP 4 kDa protein Mg2+-independent K1/2 Ca2+ ↑ [124] (bovine brain) Ca2+-ATPase 4 kDa protein Mg2+-independent K1/2 Ca2+ ↑ [122] (goat testis) Ca2+-ATPase C opyright © 2011 SciRes. ABC
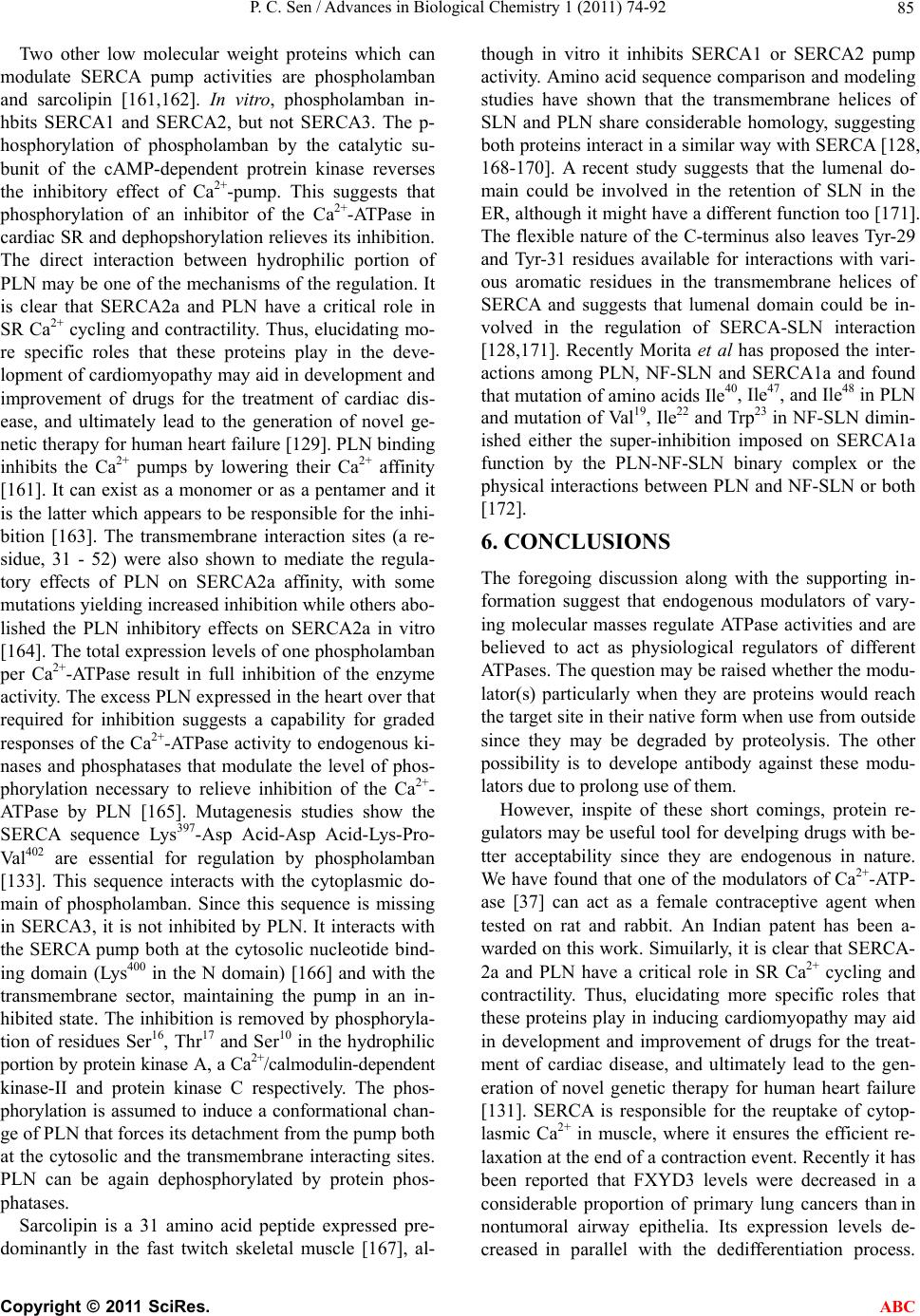 P. C. Sen / Advances in Biological Chemistry 1 (2011) 74-92 Copyright © 2011 SciRes. 85 ABC Two other low molecular weight proteins which can modulate SERCA pump activities are phospholamban and sarcolipin [161,162]. In vitro, phospholamban in- hbits SERCA1 and SERCA2, but not SERCA3. The p- hosphorylation of phospholamban by the catalytic su- bunit of the cAMP-dependent protrein kinase reverses the inhibitory effect of Ca2+-pump. This suggests that phosphorylation of an inhibitor of the Ca2+-ATPase in cardiac SR and dephopshorylation relieves its inhibition. The direct interaction between hydrophilic portion of PLN may be one of the mechanisms of the regulation. It is clear that SERCA2a and PLN have a critical role in SR Ca2+ cycling and contractility. Thus, elucidating mo- re specific roles that these proteins play in the deve- lopment of cardiomyopathy may aid in development and improvement of drugs for the treatment of cardiac dis- ease, and ultimately lead to the generation of novel ge- netic therapy for human heart failure [129]. PLN binding inhibits the Ca2+ pumps by lowering their Ca2+ affinity [161]. It can exist as a monomer or as a pentamer and it is the latter which appears to be responsible for the inhi- bition [163]. The transmembrane interaction sites (a re- sidue, 31 - 52) were also shown to mediate the regula- tory effects of PLN on SERCA2a affinity, with some mutations yielding increased inhibition while others abo- lished the PLN inhibitory effects on SERCA2a in vitro [164]. The total expression levels of one phospholamban per Ca2+-ATPase result in full inhibition of the enzyme activity. The excess PLN expressed in the heart over that required for inhibition suggests a capability for graded responses of the Ca2+-ATPase activity to endogenous ki- nases and phosphatases that modulate the level of phos- phorylation necessary to relieve inhibition of the Ca2+- ATPase by PLN [165]. Mutagenesis studies show the SERCA sequence Lys397-Asp Acid-Asp Acid-Lys-Pro- Val402 are essential for regulation by phospholamban [133]. This sequence interacts with the cytoplasmic do- main of phospholamban. Since this sequence is missing in SERCA3, it is not inhibited by PLN. It interacts with the SERCA pump both at the cytosolic nucleotide bind- ing domain (Lys400 in the N domain) [166] and with the transmembrane sector, maintaining the pump in an in- hibited state. The inhibition is removed by phosphoryla- tion of residues Ser16, Thr17 and Ser10 in the hydrophilic portion by protein kinase A, a Ca2+/calmodulin-dependent kinase-II and protein kinase C respectively. The phos- phorylation is assumed to induce a conformational chan- ge of PLN that forces its detachment from the pump both at the cytosolic and the transmembrane interacting sites. PLN can be again dephosphorylated by protein phos- phatases. Sarcolipin is a 31 amino acid peptide expressed pre- dominantly in the fast twitch skeletal muscle [167], al- though in vitro it inhibits SERCA1 or SERCA2 pump activity. Amino acid sequence comparison and modeling studies have shown that the transmembrane helices of SLN and PLN share considerable homology, suggesting both proteins interact in a similar way with SERCA [128, 168-170]. A recent study suggests that the lumenal do- main could be involved in the retention of SLN in the ER, although it might have a different function too [171]. The flexible nature of the C-terminus also leaves Tyr-29 and Tyr-31 residues available for interactions with vari- ous aromatic residues in the transmembrane helices of SERCA and suggests that lumenal domain could be in- volved in the regulation of SERCA-SLN interaction [128,171]. Recently Morita et al has proposed the inter- actions among PLN, NF-SLN and SERCA1a and found that mutation of amino acids Ile40, Ile47, and Ile48 in PLN and mutation of Val19, Ile22 and Trp23 in NF-SLN dimin- ished either the super-inhibition imposed on SERCA1a function by the PLN-NF-SLN binary complex or the physical interactions between PLN and NF-SLN or both [172]. 6. CONCLUSIONS The foregoing discussion along with the supporting in- formation suggest that endogenous modulators of vary- ing molecular masses regulate ATPase activities and are believed to act as physiological regulators of different ATPases. The question may be raised whether the modu- lator(s) particularly when they are proteins would reach the target site in their native form when use from outside since they may be degraded by proteolysis. The other possibility is to develope antibody against these modu- lators due to prolong use of them. However, inspite of these short comings, protein re- gulators may be useful tool for develping drugs with be- tter acceptability since they are endogenous in nature. We have found that one of the modulators of Ca2+-ATP- ase [37] can act as a female contraceptive agent when tested on rat and rabbit. An Indian patent has been a- warded on this work. Simuilarly, it is clear that SERCA- 2a and PLN have a critical role in SR Ca2+ cycling and contractility. Thus, elucidating more specific roles that these proteins play in inducing cardiomyopathy may aid in development and improvement of drugs for the treat- ment of cardiac disease, and ultimately lead to the gen- eration of novel genetic therapy for human heart failure [131]. SERCA is responsible for the reuptake of cytop- lasmic Ca2+ in muscle, where it ensures the efficient re- laxation at the end of a contraction event. Recently it has been reported that FXYD3 levels were decreased in a considerable proportion of primary lung cancers than in nontumoral airway epithelia. Its expression levels de- creased in parallel with the dedifferentiation process.
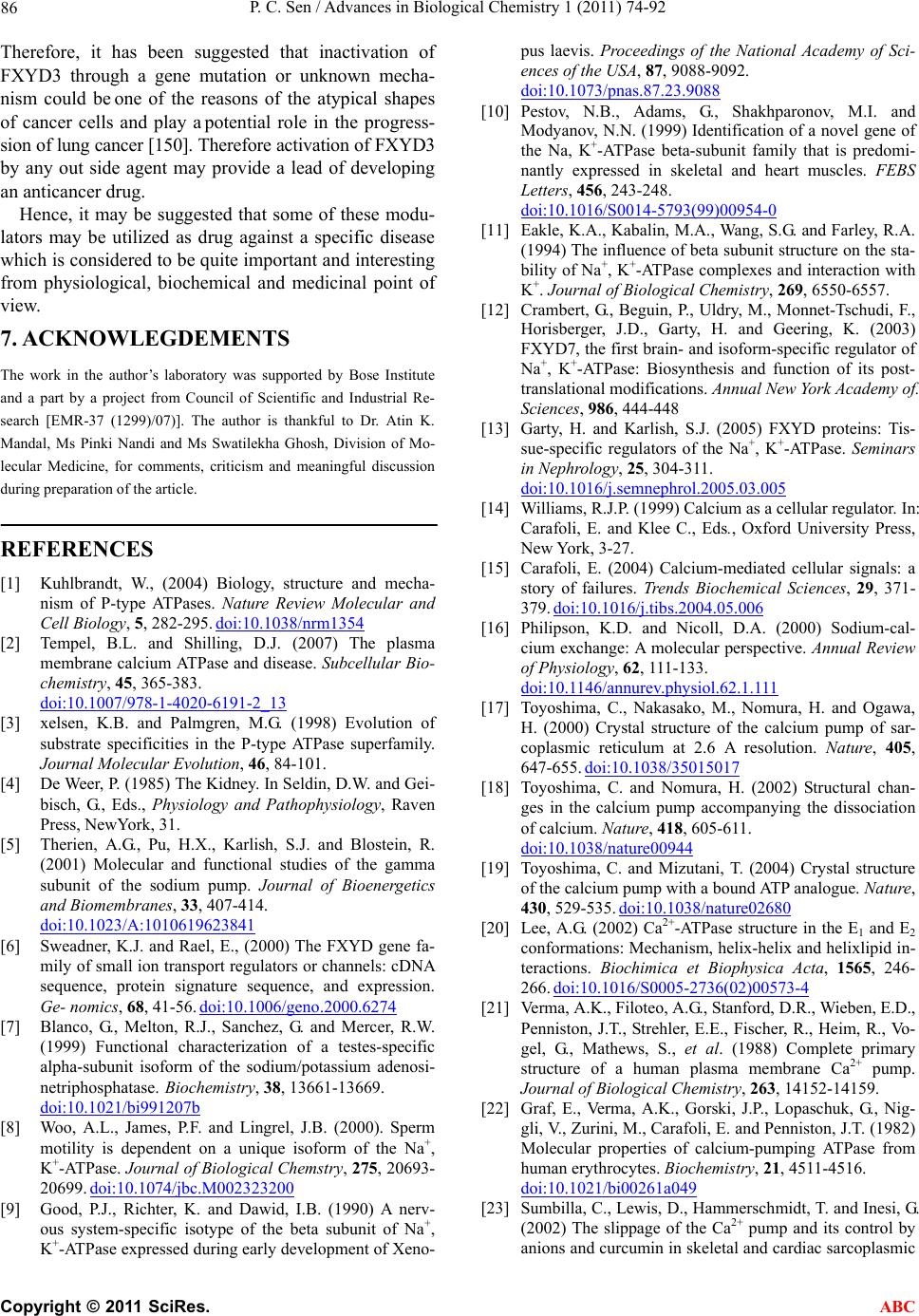 P. C. Sen / Advances in Biological Chemistry 1 (2011) 74-92 86 Therefore, it has been suggested that inactivation of FXYD3 through a gene mutation or unknown mecha- nism could be one of the reasons of the atypical shapes of cancer cells and play a potential role in the progress- sion of lung cancer [150]. Therefore activation of FXYD3 by any out side agent may provide a lead of developing an anticancer drug. Hence, it may be suggested that some of these modu- lators may be utilized as drug against a specific disease which is considered to be quite important and interesting from physiological, biochemical and medicinal point of view. 7. ACKNOWLEGDEMENTS The work in the author’s laboratory was supported by Bose Institute and a part by a project from Council of Scientific and Industrial Re- search [EMR-37 (1299)/07)]. The author is thankful to Dr. Atin K. Mandal, Ms Pinki Nandi and Ms Swatilekha Ghosh, Division of Mo- lecular Medicine, for comments, criticism and meaningful discussion during preparation of the article. REFERENCES [1] Kuhlbrandt, W., (2004) Biology, structure and mecha- nism of P-type ATPases. Nature Review Molecular and Cell Biology, 5, 282-295. doi:10.1038/nrm1354 [2] Tempel, B.L. and Shilling, D.J. (2007) The plasma membrane calcium ATPase and disease. Subcellular Bio- chemistry, 45, 365-383. doi:10.1007/978-1-4020-6191-2_13 [3] xelsen, K.B. and Palmgren, M.G. (1998) Evolution of substrate specificities in the P-type ATPase superfamily. Journal Molecular Evolution, 46, 84-101. [4] De Weer, P. (1985) The Kidney. In Seldin, D.W. and Gei- bisch, G., Eds., Physiology and Pathophysiology, Raven Press, NewYork, 31. [5] Therien, A.G., Pu, H.X., Karlish, S.J. and Blostein, R. (2001) Molecular and functional studies of the gamma subunit of the sodium pump. Journal of Bioenergetics and Biomembranes, 33, 407-414. doi:10.1023/A:1010619623841 [6] Sweadner, K.J. and Rael, E., (2000) The FXYD gene fa- mily of small ion transport regulators or channels: cDNA sequence, protein signature sequence, and expression. Ge- nomics, 68, 41-56. doi:10.1006/geno.2000.6274 [7] Blanco, G., Melton, R.J., Sanchez, G. and Mercer, R.W. (1999) Functional characterization of a testes-specific alpha-subunit isoform of the sodium/potassium adenosi- netriphosphatase. Biochemistry, 38, 13661-13669. doi:10.1021/bi991207b [8] Woo, A.L., James, P.F. and Lingrel, J.B. (2000). Sperm motility is dependent on a unique isoform of the Na+, K+-ATPase. Journal of Biological Chemstry, 275, 20693- 20699. doi:10.1074/jbc.M002323200 [9] Good, P.J., Richter, K. and Dawid, I.B. (1990) A nerv- ous system-specific isotype of the beta subunit of Na+, K+-ATPase expressed during early development of Xeno- pus laevis. Proceedings of the National Academy of Sci- ences of the USA, 87, 9088-9092. doi:10.1073/pnas.87.23.9088 [10] Pestov, N.B., Adams, G., Shakhparonov, M.I. and Modyanov, N.N. (1999) Identification of a novel gene of the Na, K+-ATPase beta-subunit family that is predomi- nantly expressed in skeletal and heart muscles. FEBS Letters, 456, 243-248. doi:10.1016/S0014-5793(99)00954-0 [11] Eakle, K.A., Kabalin, M.A., Wang, S.G. and Farley, R.A. (1994) The influence of beta subunit structure on the sta- bility of Na+, K+-ATPase complexes and interaction with K+. Journal of Biological Chemistry, 269, 6550-6557. [12] Crambert, G., Beguin, P., Uldry, M., Monnet-Tschudi, F., Horisberger, J.D., Garty, H. and Geering, K. (2003) FXYD7, the first brain- and isoform-specific regulator of Na+, K+-ATPase: Biosynthesis and function of its post- translational modifications. Annual New York Academy of. Sciences, 986, 444-448 [13] Garty, H. and Karlish, S.J. (2005) FXYD proteins: Tis- sue-specific regulators of the Na+, K+-ATPase. Seminars in Nephrology, 25, 304-311. doi:10.1016/j.semnephrol.2005.03.005 [14] Williams, R.J.P. (1999) Calcium as a cellular regulator. In: Carafoli, E. and Klee C., Eds., Oxford University Press, New York, 3-27. [15] Carafoli, E. (2004) Calcium-mediated cellular signals: a story of failures. Trends Biochemical Sciences, 29, 371- 379. doi:10.1016/j.tibs.2004.05.006 [16] Philipson, K.D. and Nicoll, D.A. (2000) Sodium-cal- cium exchange: A molecular perspective. Annual Review of Physiology, 62, 111-133. doi:10.1146/annurev.physiol.62.1.111 [17] Toyoshima, C., Nakasako, M., Nomura, H. and Ogawa, H. (2000) Crystal structure of the calcium pump of sar- coplasmic reticulum at 2.6 A resolution. Nature, 405, 647-655. doi:10.1038/35015017 [18] Toyoshima, C. and Nomura, H. (2002) Structural chan- ges in the calcium pump accompanying the dissociation of calcium. Nature, 418, 605-611. doi:10.1038/nature00944 [19] Toyoshima, C. and Mizutani, T. (2004) Crystal structure of the calcium pump with a bound ATP analogue. Nature, 430, 529-535. doi:10.1038/nature02680 [20] Lee, A.G. (2002) Ca2+-ATPase structure in the E1 and E2 conformations: Mechanism, helix-helix and helixlipid in- teractions. Biochimica et Biophysica Acta, 1565, 246- 266. doi:10.1016/S0005-2736(02)00573-4 [21] Verma, A.K., Filoteo, A.G., Stanford, D.R., Wieben, E.D., Penniston, J.T., Strehler, E.E., Fischer, R., Heim, R., Vo- gel, G., Mathews, S., et al. (1988) Complete primary structure of a human plasma membrane Ca2+ pump. Journal of Biological Chemistry, 263, 14152-14159. [22] Graf, E., Verma, A.K., Gorski, J.P., Lopaschuk, G., Nig- gli, V., Zurini, M., Carafoli, E. and Penniston, J.T. (1982) Molecular properties of calcium-pumping ATPase from human erythrocytes. Biochemistry, 21, 4511-4516. doi:10.1021/bi00261a049 [23] Sumbilla, C., Lewis, D., Hammerschmidt, T. and Inesi, G. (2002) The slippage of the Ca2+ pump and its control by anions and curcumin in skeletal and cardiac sarcoplasmic C opyright © 2011 SciRes. ABC
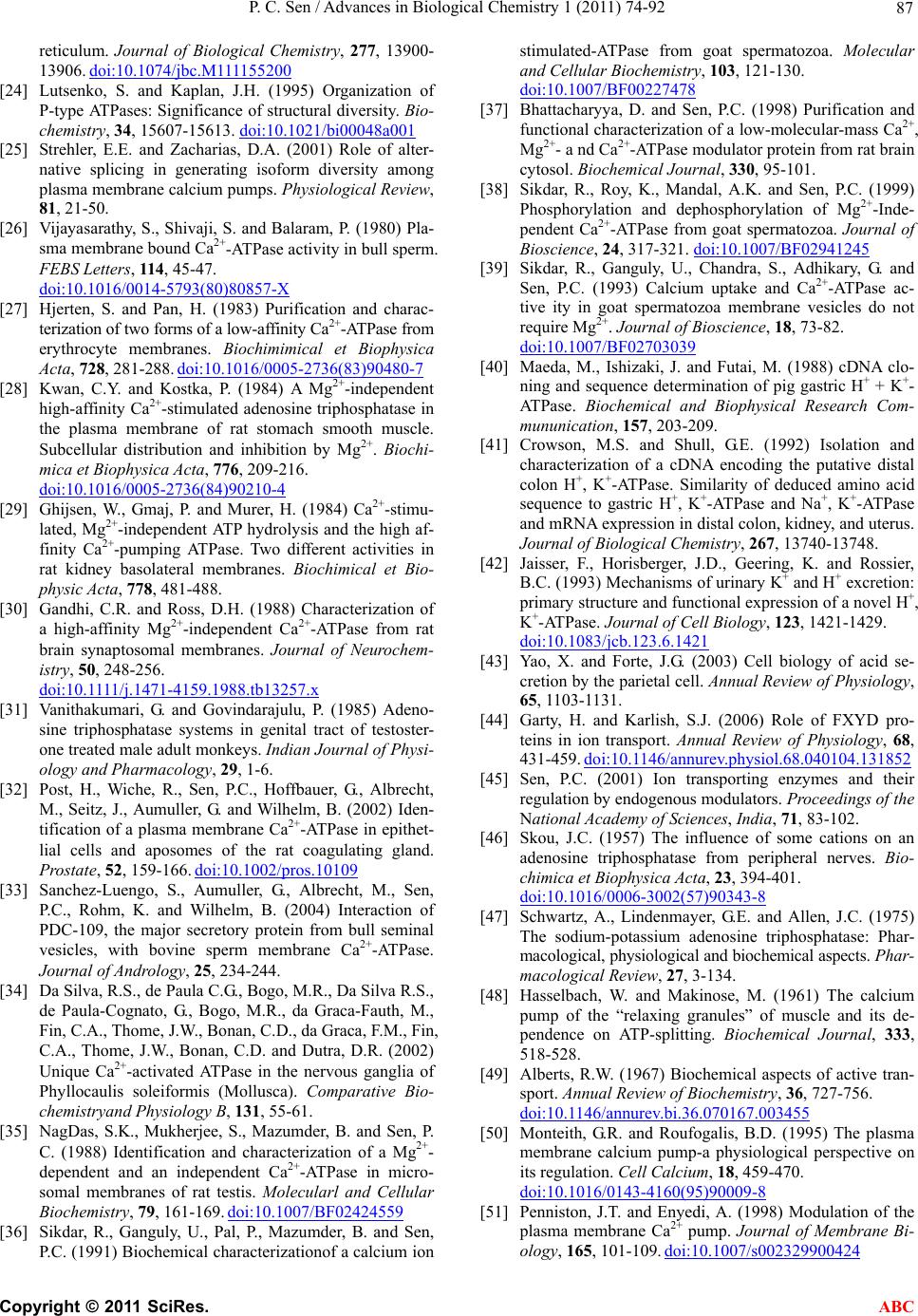 P. C. Sen / Advances in Biological Chemistry 1 (2011) 74-92 87 reticulum. Journal of Biological Chemistry, 277, 13900- 13906. doi:10.1074/jbc.M111155200 [24] Lutsenko, S. and Kaplan, J.H. (1995) Organization of P-type ATPases: Significance of structural diversity. Bio- chemistry, 34, 15607-15613. doi:10.1021/bi00048a001 [25] Strehler, E.E. and Zacharias, D.A. (2001) Role of alter- native splicing in generating isoform diversity among plasma membrane calcium pumps. Physiological Review, 81, 21-50. [26] Vijayasarathy, S., Shivaji, S. and Balaram, P. (1980) Pla- sma membrane bound Ca2+-ATPase activity in bull sperm. FEBS Letters, 114, 45-47. doi:10.1016/0014-5793(80)80857-X [27] Hjerten, S. and Pan, H. (1983) Purification and charac- terization of two forms of a low-affinity Ca2+-ATPase from erythrocyte membranes. Biochimimical et Biophysica Acta, 728, 281-288. doi:10.1016/0005-2736(83)90480-7 [28] Kwan, C.Y. and Kostka, P. (1984) A Mg2+-independent high-affinity Ca2+-stimulated adenosine triphosphatase in the plasma membrane of rat stomach smooth muscle. Subcellular distribution and inhibition by Mg2+. Biochi- mica et Biophysica Acta, 776, 209-216. doi:10.1016/0005-2736(84)90210-4 [29] Ghijsen, W., Gmaj, P. and Murer, H. (1984) Ca2+-stimu- lated, Mg2+-independent ATP hydrolysis and the high af- finity Ca2+-pumping ATPase. Two different activities in rat kidney basolateral membranes. Biochimical et Bio- physic Acta, 778, 481-488. [30] Gandhi, C.R. and Ross, D.H. (1988) Characterization of a high-affinity Mg2+-independent Ca2+-ATPase from rat brain synaptosomal membranes. Journal of Neurochem- istry, 50, 248-256. doi:10.1111/j.1471-4159.1988.tb13257.x [31] Vanithakumari, G. and Govindarajulu, P. (1985) Adeno- sine triphosphatase systems in genital tract of testoster- one treated male adult monkeys. Indian Journal of Physi- ology and Pharmacology, 29, 1-6. [32] Post, H., Wiche, R., Sen, P.C., Hoffbauer, G., Albrecht, M., Seitz, J., Aumuller, G. and Wilhelm, B. (2002) Iden- tification of a plasma membrane Ca2+-ATPase in epithet- lial cells and aposomes of the rat coagulating gland. Prostate, 52, 159-166. doi:10.1002/pros.10109 [33] Sanchez-Luengo, S., Aumuller, G., Albrecht, M., Sen, P.C., Rohm, K. and Wilhelm, B. (2004) Interaction of PDC-109, the major secretory protein from bull seminal vesicles, with bovine sperm membrane Ca2+-ATPase. Journal of Andrology, 25, 234-244. [34] Da Silva, R.S., de Paula C.G., Bogo, M.R., Da Silva R.S., de Paula-Cognato, G., Bogo, M.R., da Graca-Fauth, M., Fin, C.A., Thome, J.W., Bonan, C.D., da Graca, F.M., Fin, C.A., Thome, J.W., Bonan, C.D. and Dutra, D.R. (2002) Unique Ca2+-activated ATPase in the nervous ganglia of Phyllocaulis soleiformis (Mollusca). Comparative Bio- chemistryand Physiology B, 131, 55-61. [35] NagDas, S.K., Mukherjee, S., Mazumder, B. and Sen, P. C. (1988) Identification and characterization of a Mg2+- dependent and an independent Ca2+-ATPase in micro- somal membranes of rat testis. Molecularl and Cellular Biochemistry, 79, 161-169. doi:10.1007/BF02424559 [36] Sikdar, R., Ganguly, U., Pal, P., Mazumder, B. and Sen, P.C. (1991) Biochemical characterizationof a calcium ion stimulated-ATPase from goat spermatozoa. Molecular and Cellular Biochemistry, 103, 121-130. doi:10.1007/BF00227478 [37] Bhattacharyya, D. and Sen, P.C. (1998) Purification and functional characterization of a low-molecular-mass Ca2+, Mg2+- a nd Ca2+-ATPase modulator protein from rat brain cytosol. Biochemical Journal, 330, 95-101. [38] Sikdar, R., Roy, K., Mandal, A.K. and Sen, P.C. (1999) Phosphorylation and dephosphorylation of Mg2+-Inde- pendent Ca2+-ATPase from goat spermatozoa. Journal of Bioscience, 24, 317-321. doi:10.1007/BF02941245 [39] Sikdar, R., Ganguly, U., Chandra, S., Adhikary, G. and Sen, P.C. (1993) Calcium uptake and Ca2+-ATPase ac- tive ity in goat spermatozoa membrane vesicles do not require Mg2+. Journal of Bioscience, 18, 73-82. doi:10.1007/BF02703039 [40] Maeda, M., Ishizaki, J. and Futai, M. (1988) cDNA clo- ning and sequence determination of pig gastric H+ + K+- AT Pa se. Biochemical and Biophysical Research Com- mununication, 157, 203-209. [41] Crowson, M.S. and Shull, G.E. (1992) Isolation and characterization of a cDNA encoding the putative distal colon H+, K+-ATPase. Similarity of deduced amino acid sequence to gastric H+, K+-ATPase and Na+, K+-ATPase and mRNA expression in distal colon, kidney, and uterus. Journal of Biological Chemistry, 267, 13740-13748. [42] Jaisser, F., Horisberger, J.D., Geering, K. and Rossier, B.C. (1993) Mechanisms of urinary K+ and H+ excretion: primary structure and functional expression of a novel H+, K+-ATPase. Journal of Cell Biology, 123, 1421-1429. doi:10.1083/jcb.123.6.1421 [43] Yao, X. and Forte, J.G. (2003) Cell biology of acid se- cretion by the parietal cell. Annual Review of Physiology, 65, 1103-1131. [44] Garty, H. and Karlish, S.J. (2006) Role of FXYD pro- teins in ion transport. Annual Review of Physiology, 68, 431-459. doi:10.1146/annurev.physiol.68.040104.131852 [45] Sen, P.C. (2001) Ion transporting enzymes and their regulation by endogenous modulators. Proceedings of the National Academy of Sciences, India, 71, 83-102. [46] Skou, J.C. (1957) The influence of some cations on an adenosine triphosphatase from peripheral nerves. Bio- chimica et Biophysica Acta, 23, 394-401. doi:10.1016/0006-3002(57)90343-8 [47] Schwartz, A., Lindenmayer, G.E. and Allen, J.C. (1975) The sodium-potassium adenosine triphosphatase: Phar- macological, physiological and biochemical aspects. Phar- macological Review, 27, 3-134. [48] Hasselbach, W. and Makinose, M. (1961) The calcium pump of the “relaxing granules” of muscle and its de- pendence on ATP-splitting. Biochemical Journal, 333, 518-528. [49] Alberts, R.W. (1967) Biochemical aspects of active tran- sport. Annual Review of Biochemistry, 36, 727-756. doi:10.1146/annurev.bi.36.070167.003455 [50] Monteith, G.R. and Roufogalis, B.D. (1995) The plasma membrane calcium pump-a physiological perspective on its regulation. Cell Calcium, 18, 459-470. doi:10.1016/0143-4160(95)90009-8 [51] Penniston, J.T. and Enyedi, A. (1998) Modulation of the plasma membrane Ca2+ pump. Journal of Membrane Bi- ology, 165, 101-109. doi:10.1007/s002329900424 C opyright © 2011 SciRes. ABC
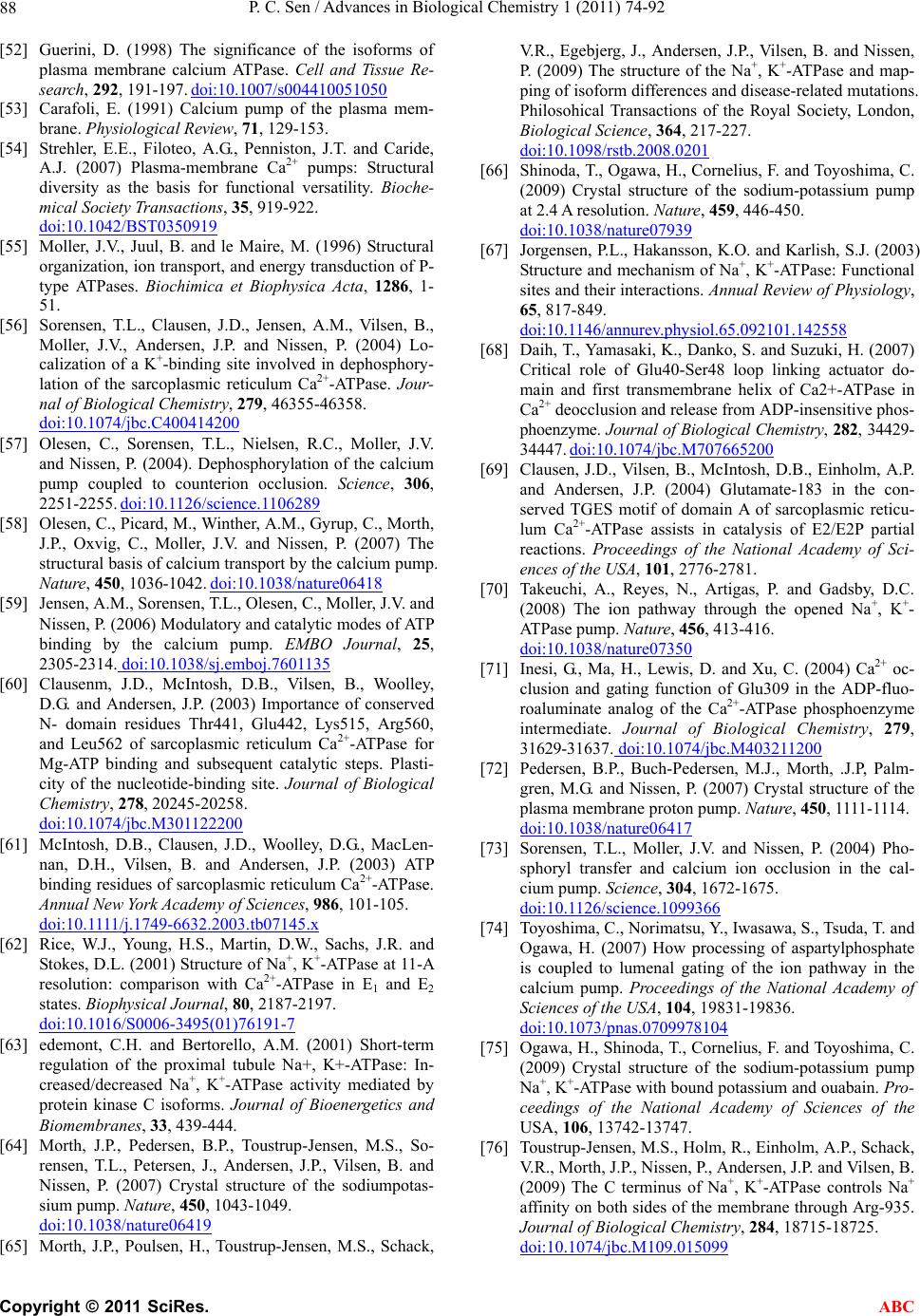 P. C. Sen / Advances in Biological Chemistry 1 (2011) 74-92 88 [52] Guerini, D. (1998) The significance of the isoforms of plasma membrane calcium ATPase. Cell and Tissue Re- search, 292, 191-197. doi:10.1007/s004410051050 [53] Carafoli, E. (1991) Calcium pump of the plasma mem- brane. Physiological Review, 71, 129-153. [54] Strehler, E.E., Filoteo, A.G., Penniston, J.T. and Caride, A.J. (2007) Plasma-membrane Ca2+ pumps: Structural diversity as the basis for functional versatility. Bioche- mical Society Transactions, 35, 919-922. doi:10.1042/BST0350919 [55] Moller, J.V., Juul, B. and le Maire, M. (1996) Structural organization, ion transport, and energy transduction of P- type ATPases. Biochimica et Biophysica Acta, 1286, 1- 51. [56] Sorensen, T.L., Clausen, J.D., Jensen, A.M., Vilsen, B., Moller, J.V., Andersen, J.P. and Nissen, P. (2004) Lo- calization of a K+-binding site involved in dephosphory- lation of the sarcoplasmic reticulum Ca2+-ATPase. Jour- nal of Biological Chemistry, 279, 46355-46358. doi:10.1074/jbc.C400414200 [57] Olesen, C., Sorensen, T.L., Nielsen, R.C., Moller, J.V. and Nissen, P. (2004). Dephosphorylation of the calcium pump coupled to counterion occlusion. Science, 306, 2251-2255. doi:10.1126/science.1106289 [58] Olesen, C., Picard, M., Winther, A.M., Gyrup, C., Morth, J.P., Oxvig, C., Moller, J.V. and Nissen, P. (2007) The structural basis of calcium transport by the calcium pump. Nature, 450, 1036-1042. doi:10.1038/nature06418 [59] Jensen, A.M., Sorensen, T.L., Olesen, C., Moller, J.V. and Nissen, P. (2006) Modulatory and catalytic modes of ATP binding by the calcium pump. EMBO Journal, 25, 2305-2314. doi:10.1038/sj.emboj.7601135 [60] Clausenm, J.D., McIntosh, D.B., Vilsen, B., Woolley, D.G. and Andersen, J.P. (2003) Importance of conserved N- domain residues Thr441, Glu442, Lys515, Arg560, and Leu562 of sarcoplasmic reticulum Ca2+-ATPase for Mg-ATP binding and subsequent catalytic steps. Plasti- city of the nucleotide-binding site. Journal of Biological Chemistry, 278, 20245-20258. doi:10.1074/jbc.M301122200 [61] McIntosh, D.B., Clausen, J.D., Woolley, D.G., MacLen- nan, D.H., Vilsen, B. and Andersen, J.P. (2003) ATP binding residues of sarcoplasmic reticulum Ca2+-ATPase. Annual New York Academy of Sciences, 986, 101-105. doi:10.1111/j.1749-6632.2003.tb07145.x [62] Rice, W.J., Young, H.S., Martin, D.W., Sachs, J.R. and Stokes, D.L. (2001) Structure of Na+, K+-ATPase at 11-A resolution: comparison with Ca2+-ATPase in E1 and E2 states. Biophysical Journal, 80, 2187-2197. doi:10.1016/S0006-3495(01)76191-7 [63] edemont, C.H. and Bertorello, A.M. (2001) Short-term regulation of the proximal tubule Na+, K+-ATPase: In- creased/decreased Na+, K+-ATPase activity mediated by protein kinase C isoforms. Journal of Bioenergetics and Biomembranes, 33, 439-444. [64] Morth, J.P., Pedersen, B.P., Toustrup-Jensen, M.S., So- rensen, T.L., Petersen, J., Andersen, J.P., Vilsen, B. and Nissen, P. (2007) Crystal structure of the sodiumpotas- sium pump. Nature, 450, 1043-1049. doi:10.1038/nature06419 [65] Morth, J.P., Poulsen, H., Toustrup-Jensen, M.S., Schack, V.R., Egebjerg, J., Andersen, J.P., Vilsen, B. and Nissen, P. (2009) The structure of the Na+, K+-ATPase and map- ping of isoform differences and disease-related mutations. Philosohical Transactions of the Royal Society, London, Biological Science, 364, 217-227. doi:10.1098/rstb.2008.0201 [66] Shinoda, T., Ogawa, H., Cornelius, F. and Toyoshima, C. (2009) Crystal structure of the sodium-potassium pump at 2.4 A resolution. Nature, 459, 446-450. doi:10.1038/nature07939 [67] Jorgensen, P.L., Hakansson, K.O. and Karlish, S.J. (2003) Structure and mechanism of Na+, K+-ATPase: Functional sites and their interactions. Annual Review of Physiology, 65, 817-849. doi:10.1146/annurev.physiol.65.092101.142558 [68] Daih, T., Yamasaki, K., Danko, S. and Suzuki, H. (2007) Critical role of Glu40-Ser48 loop linking actuator do- main and first transmembrane helix of Ca2+-ATPase in Ca2+ deocclusion and release from ADP-insensitive phos- phoenzyme. Journal of Biological Chemistry, 282, 34429- 34447. doi:10.1074/jbc.M707665200 [69] Clausen, J.D., Vilsen, B., McIntosh, D.B., Einholm, A.P. and Andersen, J.P. (2004) Glutamate-183 in the con- served TGES motif of domain A of sarcoplasmic reticu- lum Ca2+-ATPase assists in catalysis of E2/E2P partial reactions. Proceedings of the National Academy of Sci- ences of the USA, 101, 2776-2781. [70] Takeuchi, A., Reyes, N., Artigas, P. and Gadsby, D.C. (2008) The ion pathway through the opened Na+, K+- ATPase pump. Nature, 456, 413-416. doi:10.1038/nature07350 [71] Inesi, G., Ma, H., Lewis, D. and Xu, C. (2004) Ca2+ oc- clusion and gating function of Glu309 in the ADP-fluo- roaluminate analog of the Ca2+-ATPase phosphoenzyme intermediate. Journal of Biological Chemistry, 279, 31629-31637. doi:10.1074/jbc.M403211200 [72] Pedersen, B.P., Buch-Pedersen, M.J., Morth, .J.P, Palm- gren, M.G. and Nissen, P. (2007) Crystal structure of the plasma membrane proton pump. Nature, 450, 1111-1114. doi:10.1038/nature06417 [73] Sorensen, T.L., Moller, J.V. and Nissen, P. (2004) Pho- sphoryl transfer and calcium ion occlusion in the cal- cium pump. Science, 304, 1672-1675. doi:10.1126/science.1099366 [74] Toyoshima, C., Norimatsu, Y., Iwasawa, S., Tsuda, T. and Ogawa, H. (2007) How processing of aspartylphosphate is coupled to lumenal gating of the ion pathway in the calcium pump. Proceedings of the National Academy of Sciences of the USA, 104, 19831-19836. doi:10.1073/pnas.0709978104 [75] Ogawa, H., Shinoda, T., Cornelius, F. and Toyoshima, C. (2009) Crystal structure of the sodium-potassium pump Na+, K+-ATPase with bound potassium and ouabain. Pro- ceedings of the National Academy of Sciences of the USA, 106, 13742-13747. [76] Toustrup-Jensen, M.S., Holm, R., Einholm, A.P., Schack, V.R., Morth, J.P., Nissen, P., Andersen, J.P. and Vilsen, B. (2009) The C terminus of Na+, K+-ATPase controls Na+ affinity on both sides of the membrane through Arg-935. Journal of Biological Chemistry, 284, 18715-18725. doi:10.1074/jbc.M109.015099 C opyright © 2011 SciRes. ABC
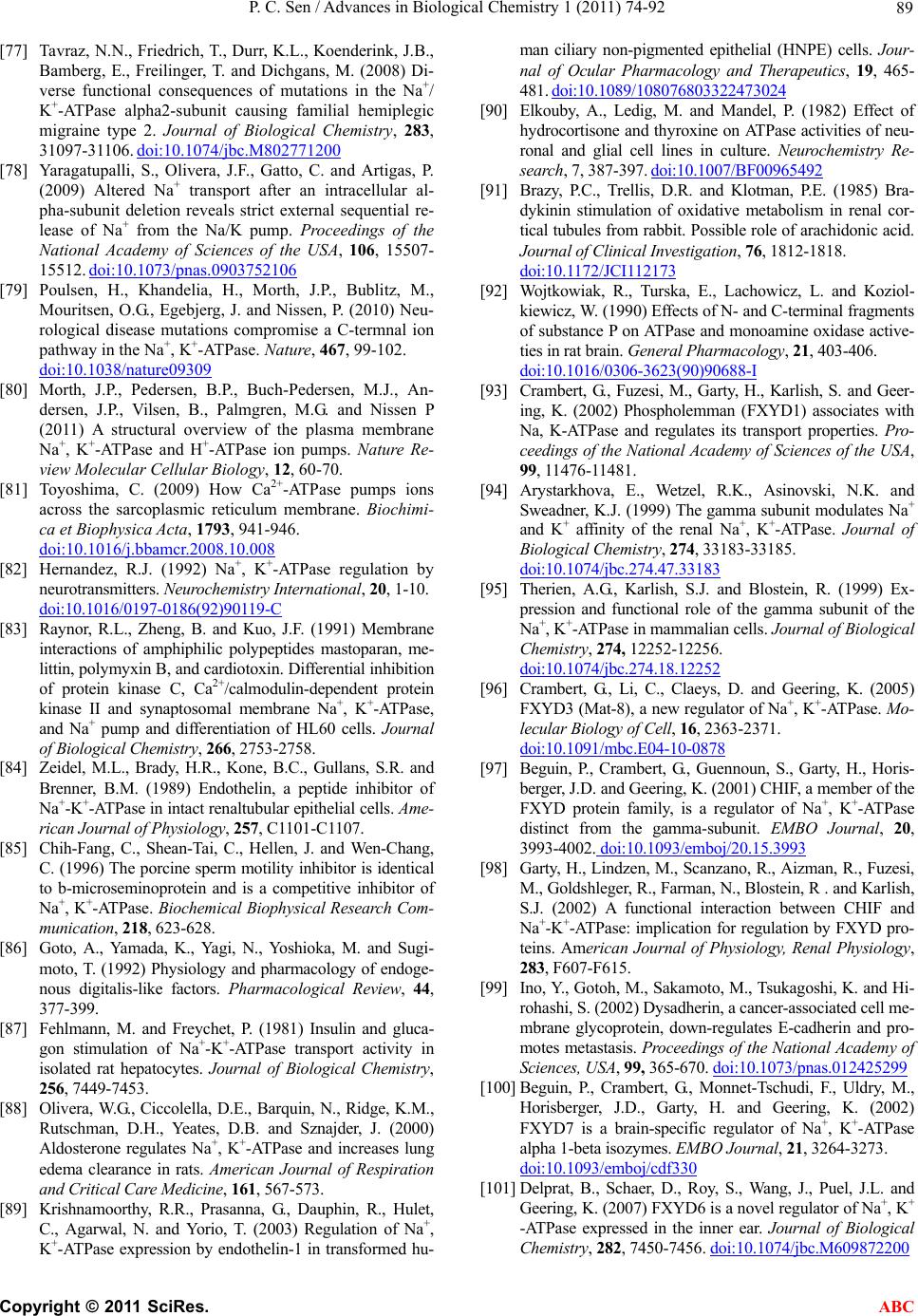 P. C. Sen / Advances in Biological Chemistry 1 (2011) 74-92 89 [77] Tavraz, N.N., Friedrich, T., Durr, K.L., Koenderink, J.B., Bamberg, E., Freilinger, T. and Dichgans, M. (2008) Di- verse functional consequences of mutations in the Na+/ K+-ATPase alpha2-subunit causing familial hemiplegic migraine type 2. Journal of Biological Chemistry, 283, 31097-31106. doi:10.1074/jbc.M802771200 [78] Yaragatupalli, S., Olivera, J.F., Gatto, C. and Artigas, P. (2009) Altered Na+ transport after an intracellular al- pha-subunit deletion reveals strict external sequential re- lease of Na+ from the Na/K pump. Proceedings of the National Academy of Sciences of the USA, 106, 15507- 15512. doi:10.1073/pnas.0903752106 [79] Poulsen, H., Khandelia, H., Morth, J.P., Bublitz, M., Mouritsen, O.G., Egebjerg, J. and Nissen, P. (2010) Neu- rological disease mutations compromise a C-termnal ion pathway in the Na+, K+-ATPase. Nature, 467, 99-102. doi:10.1038/nature09309 [80] Morth, J.P., Pedersen, B.P., Buch-Pedersen, M.J., An- dersen, J.P., Vilsen, B., Palmgren, M.G. and Nissen P (2011) A structural overview of the plasma membrane Na+, K+-ATPase and H+-ATPase ion pumps. Nature Re- view Molecular Cellular Biology, 12, 60-70. [81] Toyoshima, C. (2009) How Ca2+-ATPase pumps ions across the sarcoplasmic reticulum membrane. Biochimi- ca et Biophysica Acta, 1793, 941-946. doi:10.1016/j.bbamcr.2008.10.008 [82] Hernandez, R.J. (1992) Na+, K+-ATPase regulation by neurotransmitters. Neurochemistry International, 20, 1-10. doi:10.1016/0197-0186( 92)9 0119-C [83] Raynor, R.L., Zheng, B. and Kuo, J.F. (1991) Membrane interactions of amphiphilic polypeptides mastoparan, me- littin, polymyxin B, and cardiotoxin. Differential inhibition of protein kinase C, Ca2+/calmodulin-dependent protein kinase II and synaptosomal membrane Na+, K+-ATPase, and Na+ pump and differentiation of HL60 cells. Journal of Biological Chemistry, 266, 2753-2758. [84] Zeidel, M.L., Brady, H.R., Kone, B.C., Gullans, S.R. and Brenner, B.M. (1989) Endothelin, a peptide inhibitor of Na+-K+-ATPase in intact renaltubular epithelial cells. Ame- rican Journal of Physiology, 257, C1101-C1107. [85] Chih-Fang, C., Shean-Tai, C., Hellen, J. and Wen-Chang, C. (1996) The porcine sperm motility inhibitor is identical to b-microseminoprotein and is a competitive inhibitor of Na+, K+-ATPase. Biochemical Biophysical Research Com- munication, 218, 623-628. [86] Goto, A., Yamada, K., Yagi, N., Yoshioka, M. and Sugi- moto, T. (1992) Physiology and pharmacology of endoge- nous digitalis-like factors. Pharmacological Review, 44, 377-399. [87] Fehlmann, M. and Freychet, P. (1981) Insulin and gluca- gon stimulation of Na+-K+-ATPase transport activity in isolated rat hepatocytes. Journal of Biological Chemistry, 256, 7449-7453. [88] Olivera, W.G., Ciccolella, D.E., Barquin, N., Ridge, K.M., Rutschman, D.H., Yeates, D.B. and Sznajder, J. (2000) Aldosterone regulates Na+, K+-ATPase and increases lung edema clearance in rats. American Journal of Respiration and Critical Care Medicine, 161, 567-573. [89] Krishnamoorthy, R.R., Prasanna, G., Dauphin, R., Hulet, C., Agarwal, N. and Yorio, T. (2003) Regulation of Na+, K+-ATPase expression by endothelin-1 in transformed hu- man ciliary non-pigmented epithelial (HNPE) cells. Jour- nal of Ocular Pharmacology and Therapeutics, 19, 465- 481. doi:10.1089/1080 768033 2247302 4 [90] Elkouby, A., Ledig, M. and Mandel, P. (1982) Effect of hydrocortisone and thyroxine on ATPase activities of neu- ronal and glial cell lines in culture. Neurochemistry Re- search, 7, 387-397. doi:10.1007/BF00965492 [91] Brazy, P.C., Trellis, D.R. and Klotman, P.E. (1985) Bra- dykinin stimulation of oxidative metabolism in renal cor- tical tubules from rabbit. Possible role of arachidonic acid. Journal of Clinical Investigation, 76, 1812-1818. doi:10.1172/JCI112173 [92] Wojtkowiak, R., Turska, E., Lachowicz, L. and Koziol- kiewicz, W. (1990) Effects of N- and C-terminal fragments of substance P on ATPase and monoamine oxidase active- ties in rat brain. General Pharmacology, 21, 403-406. doi:10.1016/0306-3623( 90)9 0688-I [93] Crambert, G., Fuzesi, M., Garty, H., Karlish, S. and Geer- ing, K. (2002) Phospholemman (FXYD1) associates with Na, K-ATPase and regulates its transport properties. Pro- ceedings of the National Academy of Sciences of the USA, 99, 11476-11481. [94] Arystarkhova, E., Wetzel, R.K., Asinovski, N.K. and Sweadner, K.J. (1999) The gamma subunit modulates Na+ and K+ affinity of the renal Na+, K+-ATPase. Journal of Biological Chemistry, 274, 33183-33185. doi:10.1074/jbc.274.47. 33183 [95] Therien, A.G., Karlish, S.J. and Blostein, R. (1999) Ex- pression and functional role of the gamma subunit of the Na+, K+-ATPase in mammalian cells. Journal of Biological Chemistry, 274, 12252-12256. doi:10.1074/jbc.274.18. 12252 [96] Crambert, G., Li, C., Claeys, D. and Geering, K. (2005) FXYD3 (Mat-8), a new regulator of Na+, K+-ATPase. Mo- lecular Biology of Cell, 16, 2363-2371. doi:10.1091/mbc.E04-10 -0878 [97] Beguin, P., Crambert, G., Guennoun, S., Garty, H., Horis- berger, J.D. and Geering, K. (2001) CHIF, a member of the FXYD protein family, is a regulator of Na+, K+-ATPase distinct from the gamma-subunit. EMBO Journal, 20, 3993-4002. doi:10.1093/e mboj/20 .15.399 3 [98] Garty, H., Lindzen, M., Scanzano, R., Aizman, R., Fuzesi, M., Goldshleger, R., Farman, N., Blostein, R . and Karlish, S.J. (2002) A functional interaction between CHIF and Na+-K+-ATPase: implication for regulation by FXYD pro- teins. American Journal of Physiology, Renal Physiology, 283, F607-F615. [99] Ino, Y., Gotoh, M., Sakamoto, M., Tsukagoshi, K. and Hi- rohashi, S. (2002) Dysadherin, a cancer-associated cell me- mbrane glycoprotein, down-regulates E-cadherin and pro- motes metastasis. Proceedings of the National Academy of Sciences, USA, 99, 365-670. doi:10.1073/pnas.0124252 99 [100] Beguin, P., Crambert, G., Monnet-Tschudi, F., Uldry, M., Horisberger, J.D., Garty, H. and Geering, K. (2002) FXYD7 is a brain-specific regulator of Na+, K+-ATPase alpha 1-beta isozymes. EMBO Journal, 21, 3264-3273. doi:10.1093/emboj/cdf330 [101] Delprat, B., Schaer, D., Roy, S., Wang, J., Puel, J.L. and Geering, K. (2007) FXYD6 is a novel regulator of Na+, K+ -ATPase expressed in the inner ear. Journal of Biological Chemistry, 282, 7450-7456. doi:10.1074/j bc.M6098722 00 C opyright © 2011 SciRes. ABC
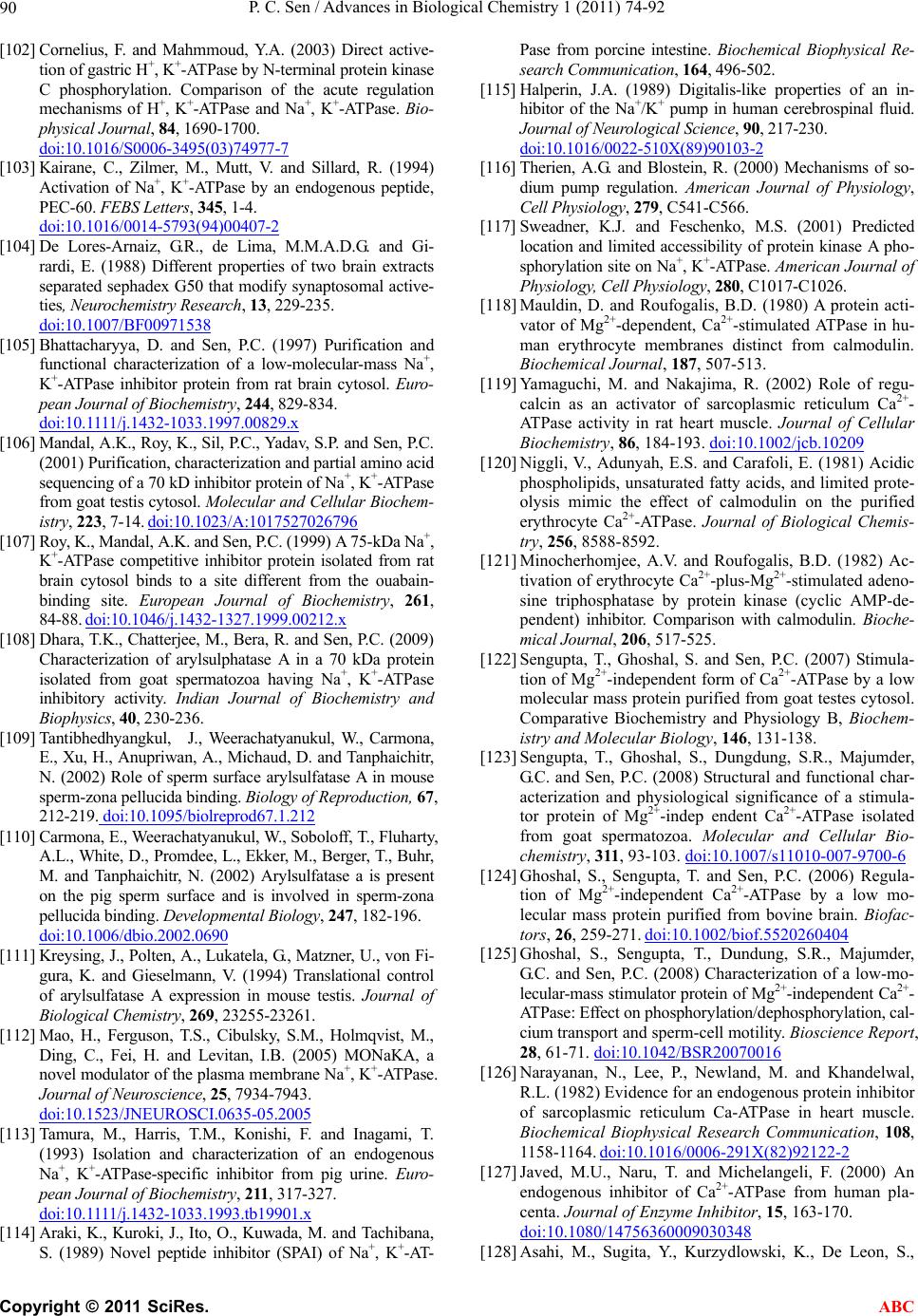 P. C. Sen / Advances in Biological Chemistry 1 (2011) 74-92 90 [102] Cornelius, F. and Mahmmoud, Y.A. (2003) Direct active- tion of gastric H+, K+-ATPase by N-terminal protein kinase C phosphorylation. Comparison of the acute regulation mechanisms of H+, K+-ATPase and Na+, K+-ATPase. Bio- physical Journal, 84, 1690-1700. doi:10.1016/S0006-3495 (03) 74977-7 [103] Kairane, C., Zilmer, M., Mutt, V. and Sillard, R. (1994) Activation of Na+, K+-ATPase by an endogenous peptide, PEC-60. FEBS Letters, 345, 1-4. doi:10.1016/0014-5793( 94)0 0407-2 [104] De Lores-Arnaiz, G.R., de Lima, M.M.A.D.G. and Gi- rardi, E. (1988) Different properties of two brain extracts separated sephadex G50 that modify synaptosomal active- ties, Neurochemistry Research, 13, 229-235. doi:10.1007/BF00971 538 [105] Bhattacharyya, D. and Sen, P.C. (1997) Purification and functional characterization of a low-molecular-mass Na+, K+-ATPase inhibitor protein from rat brain cytosol. Euro- pean Journal of Biochemistry, 244, 829-834. doi:10.1111/j.1432-1033.1997.0 0829.x [106] Mandal, A.K., Roy, K., Sil, P.C., Yadav, S.P. and Sen, P.C. (2001) Purification, characterization and partial amino acid sequencing of a 70 kD inhibitor protein of Na+, K+-ATPase from goat testis cytosol. Molecular and Cellular Biochem- istry, 223, 7-14. doi:10.1023/A:10175270267 96 [107] Roy, K., Mandal, A.K. and Sen, P.C. (1999) A 75-kDa Na+, K+-ATPase competitive inhibitor protein isolated from rat brain cytosol binds to a site different from the ouabain- binding site. European Journal of Biochemistry, 261, 84-88. doi:10.1046/j.1432-1 327. 1999.00 212. x [108] Dhara, T.K., Chatterjee, M., Bera, R. and Sen, P.C. (2009) Characterization of arylsulphatase A in a 70 kDa protein isolated from goat spermatozoa having Na+, K+-ATPase inhibitory activity. Indian Journal of Biochemistry and Biophysics, 40, 230-236. [109] Tantibhedhyangkul, J., Weerachatyanukul, W., Carmona, E., Xu, H., Anupriwan, A., Michaud, D. and Tanphaichitr, N. (2002) Role of sperm surface arylsulfatase A in mouse sperm-zona pellucida binding. Biology of Reproduction, 67, 212-219. doi:10.1095/biolre prod6 7.1.212 [110] Carmona, E., Weerachatyanukul, W., Soboloff, T., Fluharty, A.L., White, D., Promdee, L., Ekker, M., Berger, T., Buhr, M. and Tanphaichitr, N. (2002) Arylsulfatase a is present on the pig sperm surface and is involved in sperm-zona pellucida binding. Developmental Biology, 247, 182-196. doi:10.1006/dbio.2002.0 690 [111] Kreysing, J., Polten, A., Lukatela, G., Matzner, U., von Fi- gura, K. and Gieselmann, V. (1994) Translational control of arylsulfatase A expression in mouse testis. Journal of Biological Chemistry, 269, 23255-23261. [112] Mao, H., Ferguson, T.S., Cibulsky, S.M., Holmqvist, M., Ding, C., Fei, H. and Levitan, I.B. (2005) MONaKA, a novel modulator of the plasma membrane Na+, K+-ATPase. Journal of Neuroscience, 25, 7934-7943. doi:10.1523/JNEUROSCI.0 635-0 5.2005 [113] Tamura, M., Harris, T.M., Konishi, F. and Inagami, T. (1993) Isolation and characterization of an endogenous Na+, K+-ATPase-specific inhibitor from pig urine. Euro- pean Journal of Biochemistry, 211, 317-327. doi:10.1111/j.1432-1033.1993.tb 19901.x [114] Araki, K., Kuroki, J., Ito, O., Kuwada, M. and Tachibana, S. (1989) Novel peptide inhibitor (SPAI) of Na+, K+-AT- Pase from porcine intestine. Biochemical Biophysical Re- search Communication, 164, 496-502. [115] Halperin, J.A. (1989) Digitalis-like properties of an in- hibitor of the Na+/K+ pump in human cerebrospinal fluid. Journal of Neurological Science, 90, 217-230. doi:10.1016/0022-510X(89) 9010 3-2 [116] Therien, A.G. and Blostein, R. (2000) Mechanisms of so- dium pump regulation. American Journal of Physiology, Cell Physiology, 279, C541-C566. [117] Sweadner, K.J. and Feschenko, M.S. (2001) Predicted location and limited accessibility of protein kinase A pho- sphorylation site on Na+, K+-ATPase. American Journal of Physiology, Cell Physiology, 280, C1017-C1026. [118] Mauldin, D. and Roufogalis, B.D. (1980) A protein acti- vator of Mg2+-dependent, Ca2+-stimulated ATPase in hu- man erythrocyte membranes distinct from calmodulin. Biochemical Journal, 187, 507-513. [119] Yamaguchi, M. and Nakajima, R. (2002) Role of regu- calcin as an activator of sarcoplasmic reticulum Ca2+- ATPase activity in rat heart muscle. Journal of Cellular Biochemistry, 86, 184-193. doi:10.1002/jcb.10209 [120] Niggli, V., Adunyah, E.S. and Carafoli, E. (1981) Acidic phospholipids, unsaturated fatty acids, and limited prote- olysis mimic the effect of calmodulin on the purified erythrocyte Ca2+-ATPase. Journal of Biological Chemis- try, 256, 8588-8592. [121] Minocherhomjee, A.V. and Roufogalis, B.D. (1982) Ac- tivation of erythrocyte Ca2+-plus-Mg2+-stimulated adeno- sine triphosphatase by protein kinase (cyclic AMP-de- pendent) inhibitor. Comparison with calmodulin. Bioche- mical Journal, 206, 517-525. [122] Sengupta, T., Ghoshal, S. and Sen, P.C. (2007) Stimula- tion of Mg2+-independent form of Ca2+-ATPase by a low molecular mass protein purified from goat testes cytosol. Comparative Biochemistry and Physiology B, Biochem- istry and Molecular Biology, 146, 131-138. [123] Sengupta, T., Ghoshal, S., Dungdung, S.R., Majumder, G.C. and Sen, P.C. (2008) Structural and functional char- acterization and physiological significance of a stimula- tor protein of Mg2+-indep endent Ca2+-ATPase isolated from goat spermatozoa. Molecular and Cellular Bio- chemistry, 311, 93-103. doi:10.1007/s11010-007-9700-6 [124] Ghoshal, S., Sengupta, T. and Sen, P.C. (2006) Regula- tion of Mg2+-independent Ca2+-ATPase by a low mo- lecular mass protein purified from bovine brain. Biofac- tors, 26, 259-271. doi:10.1002/biof.5520260404 [125] Ghoshal, S., Sengupta, T., Dundung, S.R., Majumder, G.C. and Sen, P.C. (2008) Characterization of a low-mo- lecular-mass stimulator protein of Mg2+-independent Ca2+- ATPase: Effect on phosphorylation/dephosphorylation, cal- cium transport and sperm-cell motility. Bioscience Report, 28, 61-71. doi:10.1042/BSR20070016 [126] Narayanan, N., Lee, P., Newland, M. and Khandelwal, R.L. (1982) Evidence for an endogenous protein inhibitor of sarcoplasmic reticulum Ca-ATPase in heart muscle. Biochemical Biophysical Research Communication, 108, 1158-1164. doi:10.1016/0006-291X(82)92122-2 [127] Javed, M.U., Naru, T. and Michelangeli, F. (2000) An endogenous inhibitor of Ca2+-ATPase from human pla- centa. Journal of Enzyme Inhibitor, 15, 163-170. doi:10.1080/14756360009030348 [128] Asahi, M., Sugita, Y., Kurzydlowski, K., De Leon, S., C opyright © 2011 SciRes. ABC
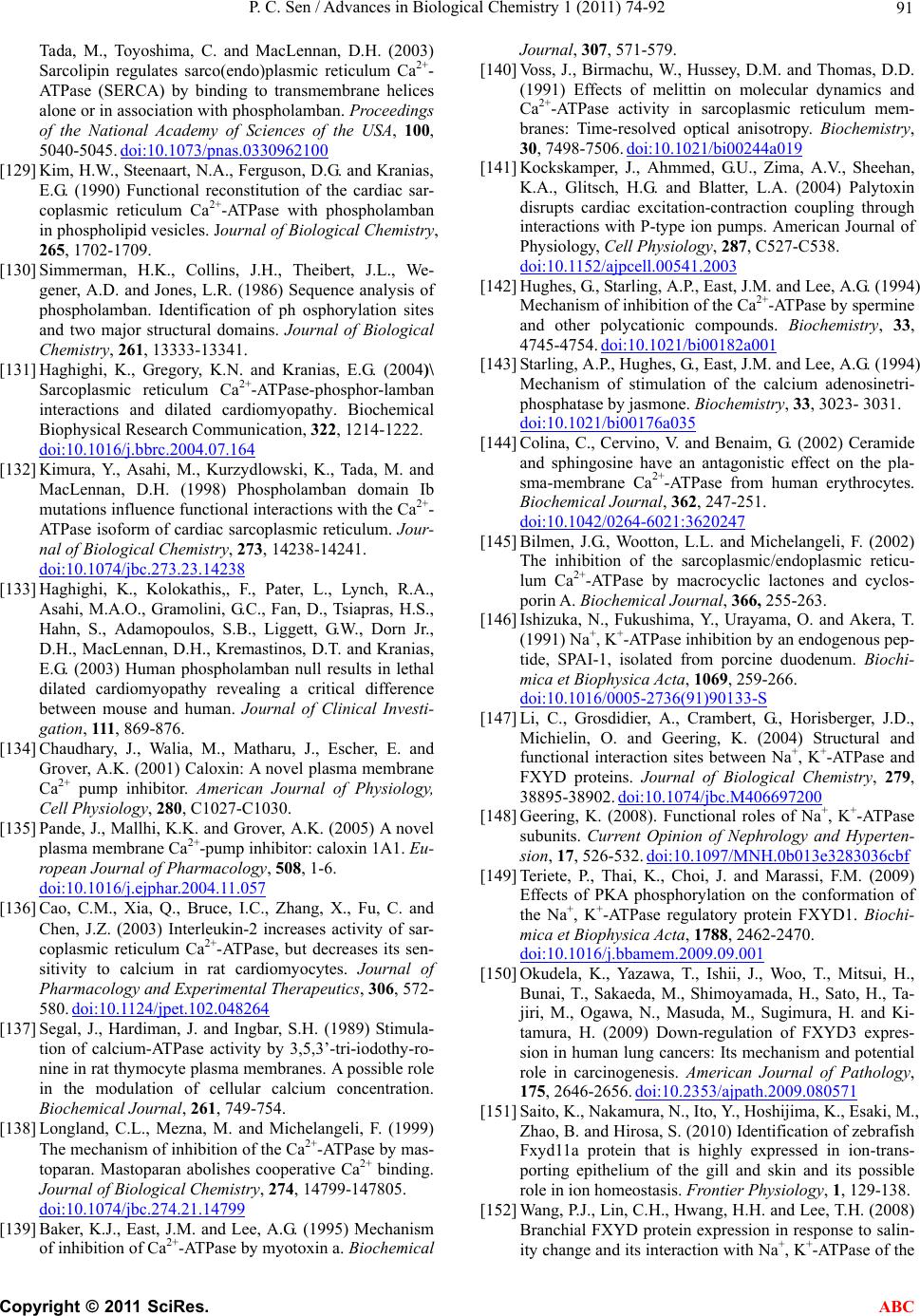 P. C. Sen / Advances in Biological Chemistry 1 (2011) 74-92 91 Tada, M., Toyoshima, C. and MacLennan, D.H. (2003) Sarcolipin regulates sarco(endo)plasmic reticulum Ca2+- ATPase (SERCA) by binding to transmembrane helices alone or in association with phospholamban. Proceedings of the National Academy of Sciences of the USA, 100, 5040-5045. doi:10.1073/pnas.0330962100 [129] Kim, H.W., Steenaart, N.A., Ferguson, D.G. and Kranias, E.G. (1990) Functional reconstitution of the cardiac sar- coplasmic reticulum Ca2+-ATPase with phospholamban in phospholipid vesicles. Journal of Biological Chemistry, 265, 1702-1709. [130] Simmerman, H.K., Collins, J.H., Theibert, J.L., We- gener, A.D. and Jones, L.R. (1986) Sequence analysis of phospholamban. Identification of ph osphorylation sites and two major structural domains. Journal of Biological Chemistry, 261, 13333-13341. [131] Haghighi, K., Gregory, K.N. and Kranias, E.G. (2004)\ Sarcoplasmic reticulum Ca2+-ATPase-phosphor-lamban interactions and dilated cardiomyopathy. Biochemical Biophysical Research Communication, 322, 1214-1222. doi:10.1016/j.bbrc.2004.07.164 [132] Kimura, Y., Asahi, M., Kurzydlowski, K., Tada, M. and MacLennan, D.H. (1998) Phospholamban domain Ib mutations influence functional interactions with the Ca2+- ATPase isoform of cardiac sarcoplasmic reticulum. Jour- nal of Biological Chemistry, 273, 14238-14241. doi:10.1074/jbc.273.23.14238 [133] Haghighi, K., Kolokathis,, F., Pater, L., Lynch, R.A., Asahi, M.A.O., Gramolini, G.C., Fan, D., Tsiapras, H.S., Hahn, S., Adamopoulos, S.B., Liggett, G.W., Dorn Jr., D.H., MacLennan, D.H., Kremastinos, D.T. and Kranias, E.G. (2003) Human phospholamban null results in lethal dilated cardiomyopathy revealing a critical difference between mouse and human. Journal of Clinical Investi- gation, 111, 869-876. [134] Chaudhary, J., Walia, M., Matharu, J., Escher, E. and Grover, A.K. (2001) Caloxin: A novel plasma membrane Ca2+ pump inhibitor. American Journal of Physiology, Cell Physiology, 280, C1027-C1030. [135] Pande, J., Mallhi, K.K. and Grover, A.K. (2005) A novel plasma membrane Ca2+-pump inhibitor: caloxin 1A1. Eu- ropean Journal of Pharmacology, 508, 1-6. doi:10.1016/j.ejphar.2004.11.057 [136] Cao, C.M., Xia, Q., Bruce, I.C., Zhang, X., Fu, C. and Chen, J.Z. (2003) Interleukin-2 increases activity of sar- coplasmic reticulum Ca2+-ATPase, but decreases its sen- sitivity to calcium in rat cardiomyocytes. Journal of Pharmacology and Experimental Therapeutics, 306, 572- 580. doi:10.1124/jpet.102.048264 [137] Segal, J., Hardiman, J. and Ingbar, S.H. (1989) Stimula- tion of calcium-ATPase activity by 3,5,3’-tri-iodothy-ro- nine in rat thymocyte plasma membranes. A possible role in the modulation of cellular calcium concentration. Biochemical Journal, 261, 749-754. [138] Longland, C.L., Mezna, M. and Michelangeli, F. (1999) The mechanism of inhibition of the Ca2+-ATPase by mas- toparan. Mastoparan abolishes cooperative Ca2+ binding. Journal of Biological Chemistry, 274, 14799-147805. doi:10.1074/jbc.274.21.14799 [139] Baker, K.J., East, J.M. and Lee, A.G. (1995) Mechanism of inhibition of Ca2+-ATPase by myotoxin a. Biochemical Journal, 307, 571-579. [140] Voss, J., Birmachu, W., Hussey, D.M. and Thomas, D.D. (1991) Effects of melittin on molecular dynamics and Ca2+-ATPase activity in sarcoplasmic reticulum mem- branes: Time-resolved optical anisotropy. Bioche mistry, 30, 7498-7506. doi:10.1021/bi00244a019 [141] Kockskamper, J., Ahmmed, G.U., Zima, A.V., Sheehan, K.A., Glitsch, H.G. and Blatter, L.A. (2004) Palytoxin disrupts cardiac excitation-contraction coupling through interactions with P-type ion pumps. American Journal of Physiology, Cell Physiology, 287, C527-C538. doi:10.1152/ajpcell.00541.2003 [142] Hughes, G., Starling, A.P., East, J.M. and Lee, A.G. (1994) Mechanism of inhibition of the Ca2+-ATPase by spermine and other polycationic compounds. Biochemistry, 33, 4745-4754. doi:10.1021/bi00182a001 [143] Starling, A.P., Hughes, G., East, J.M. and Lee, A.G. (1994) Mechanism of stimulation of the calcium adenosinetri- phosphatase by jasmone. Biochemistry, 33, 3023- 3031. doi:10.1021/bi00176a035 [144] Colina, C., Cervino, V. and Benaim, G. (2002) Ceramide and sphingosine have an antagonistic effect on the pla- sma-membrane Ca2+-ATPase from human erythrocytes. Biochemical Journal, 362, 247-251. doi:10.1042/0264-6021:3620247 [145] Bilmen, J.G., Wootton, L.L. and Michelangeli, F. (2002) The inhibition of the sarcoplasmic/endoplasmic reticu- lum Ca2+-ATPase by macrocyclic lactones and cyclos- porin A. Biochemical Journal, 366, 255-263. [146] Ishizuka, N., Fukushima, Y., Urayama, O. and Akera, T. (1991) Na+, K+-ATPase inhibition by an endogenous pep- tide, SPAI-1, isolated from porcine duodenum. Biochi- mica et Biophysica Acta, 1069, 259-266. doi:10.1016/0005-2736(91)90133-S [147] Li, C., Grosdidier, A., Crambert, G., Horisberger, J.D., Michielin, O. and Geering, K. (2004) Structural and functional interaction sites between Na+, K+-ATPase and FXYD proteins. Journal of Biological Chemistry, 279, 38895-38902. doi:10.1074/jbc.M406697200 [148] Geering, K. (2008). Functional roles of Na+, K+-ATPase subunits. Current Opinion of Nephrology and Hyperten- sion, 17, 526-532. doi:10.1097/MNH.0b013e3283036cbf [149] Teriete, P., Thai, K., Choi, J. and Marassi, F.M. (2009) Effects of PKA phosphorylation on the conformation of the Na+, K+-ATPase regulatory protein FXYD1. Biochi- mica et Biophysica Acta, 1788, 2462-2470. doi:10.1016/j.bbamem.2009.09.001 [150] Okudela, K., Yazawa, T., Ishii, J., Woo, T., Mitsui, H., Bunai, T., Sakaeda, M., Shimoyamada, H., Sato, H., Ta- jiri, M., Ogawa, N., Masuda, M., Sugimura, H. and Ki- tamura, H. (2009) Down-regulation of FXYD3 expres- sion in human lung cancers: Its mechanism and potential role in carcinogenesis. American Journal of Pathology, 175, 2646-2656. doi:10.2353/ajpath.2009.080571 [151] Saito, K., Nakamura, N., Ito, Y., Hoshijima, K., Esaki, M., Zhao, B. and Hirosa, S. (2010) Identification of zebrafish Fxyd11a protein that is highly expressed in ion-trans- porting epithelium of the gill and skin and its possible role in ion homeostasis. Frontier Physiology, 1, 129-138. [152] Wang, P.J., Lin, C.H., Hwang, H.H. and Lee, T.H. (2008) Branchial FXYD protein expression in response to salin- ity change and its interaction with Na+, K+-ATPase of the C opyright © 2011 SciRes. ABC
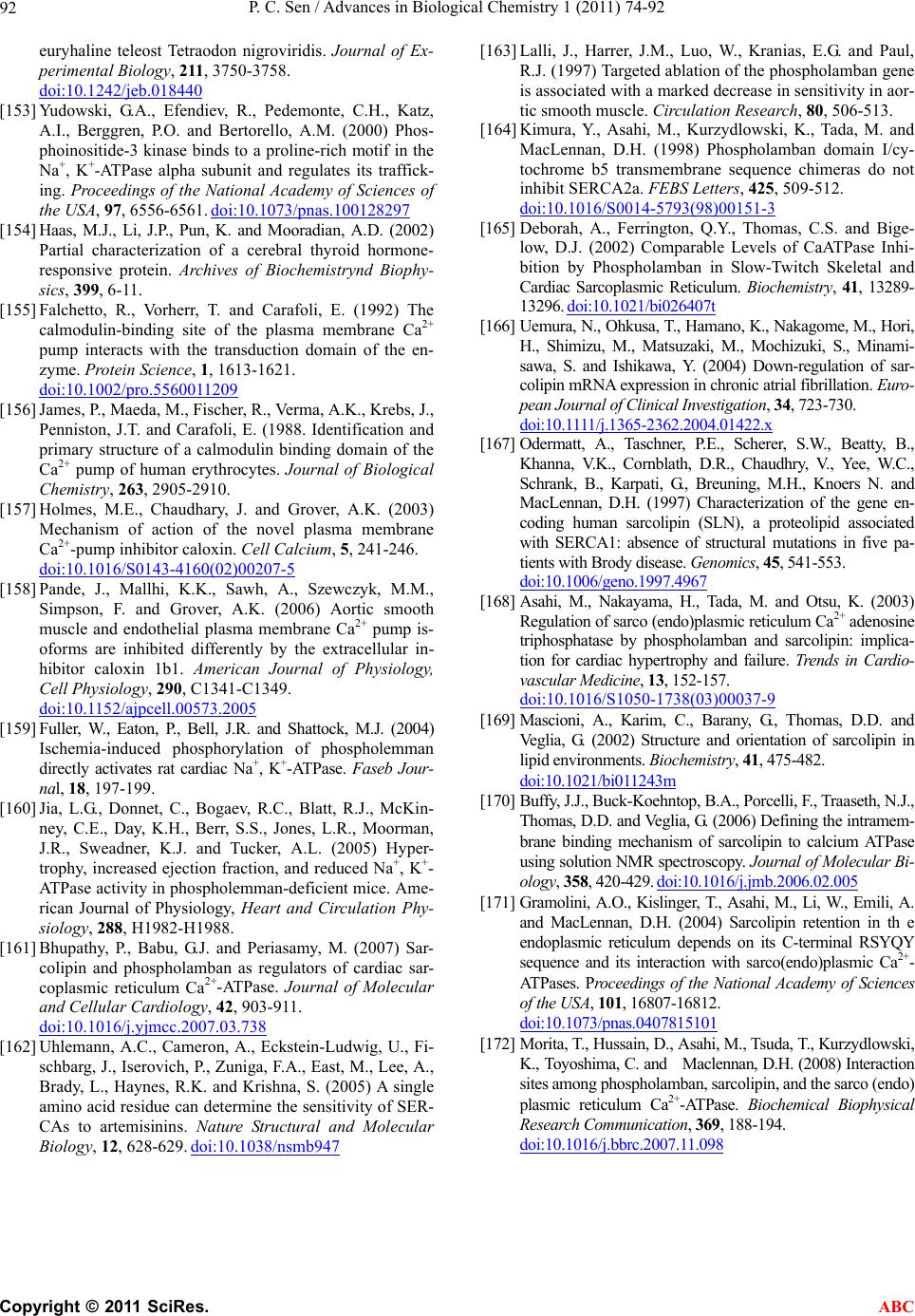 P. C. Sen / Advances in Biological Chemistry 1 (2011) 74-92 Copyright © 2011 SciRes. 92 ABC euryhaline teleost Tetraodon nigroviridis. Journal of Ex- perimental Biology, 211, 3750-3758. doi:10.1242/jeb.018440 [153] Yudowski, G.A., Efendiev, R., Pedemonte, C.H., Katz, A.I., Berggren, P.O. and Bertorello, A.M. (2000) Phos- phoinositide-3 kinase binds to a proline-rich motif in the Na+, K+-ATPase alpha subunit and regulates its traffick- ing. Proceedings of the National Academy of Sciences of the USA, 97, 6556-6561. doi:10.1073/pnas.100128297 [154] Haas, M.J., Li, J.P., Pun, K. and Mooradian, A.D. (2002) Partial characterization of a cerebral thyroid hormone- responsive protein. Archives of Biochemistrynd Biophy- sics, 399, 6-11. [155] Falchetto, R., Vorherr, T. and Carafoli, E. (1992) The calmodulin-binding site of the plasma membrane Ca2+ pump interacts with the transduction domain of the en- zyme. Protein Science, 1, 1613-1621. doi:10.1002/pro.5560011209 [156] James, P., Maeda, M., Fischer, R., Verma, A.K., Krebs, J., Penniston, J.T. and Carafoli, E. (1988. Identification and primary structure of a calmodulin binding domain of the Ca2+ pump of human erythrocytes. Journal of Biological Chemistry, 263, 2905-2910. [157] Holmes, M.E., Chaudhary, J. and Grover, A.K. (2003) Mechanism of action of the novel plasma membrane Ca2+-pump inhibitor caloxin. Cell Calcium, 5, 241-246. doi:10.1016/S0143-4160(02)00207-5 [158] Pande, J., Mallhi, K.K., Sawh, A., Szewczyk, M.M., Simpson, F. and Grover, A.K. (2006) Aortic smooth muscle and endothelial plasma membrane Ca2+ pump is- oforms are inhibited differently by the extracellular in- hibitor caloxin 1b1. American Journal of Physiology, Cell Physiology, 290, C1341-C1349. doi:10.1152/ajpcell.00573.2005 [159] Fuller, W., Eaton, P., Bell, J.R. and Shattock, M.J. (2004) Ischemia-induced phosphorylation of phospholemman directly activates rat cardiac Na+, K+-ATPase. Faseb Jour- nal, 18, 197-199. [160] Jia, L.G., Donnet, C., Bogaev, R.C., Blatt, R.J., McKin- ney, C.E., Day, K.H., Berr, S.S., Jones, L.R., Moorman, J.R., Sweadner, K.J. and Tucker, A.L. (2005) Hyper- trophy, increased ejection fraction, and reduced Na+, K+- ATPase activity in phospholemman-deficient mice. Ame- rican Journal of Physiology, Heart and Circulation Phy- siology, 288, H1982-H1988. [161] Bhupathy, P., Babu, G.J. and Periasamy, M. (2007) Sar- colipin and phospholamban as regulators of cardiac sar- coplasmic reticulum Ca2+-ATPase. Journal of Molecular and Cellular Cardiology, 42, 903-911. doi:10.1016/j.yjmcc.2007.03.738 [162] Uhlemann, A.C., Cameron, A., Eckstein-Ludwig, U., Fi- schbarg, J., Iserovich, P., Zuniga, F.A., East, M., Lee, A., Brady, L., Haynes, R.K. and Krishna, S. (2005) A single amino acid residue can determine the sensitivity of SER- CAs to artemisinins. Nature Structural and Molecular Biology, 12, 628-629. doi:10.1038/nsmb947 [163] Lalli, J., Harrer, J.M., Luo, W., Kranias, E.G. and Paul, R.J. (1997) Targeted ablation of the phospholamban gene is associated with a marked decrease in sensitivity in aor- tic smooth muscle. Circulation Research, 80, 506-513. [164] Kimura, Y., Asahi, M., Kurzydlowski, K., Tada, M. and MacLennan, D.H. (1998) Phospholamban domain I/cy- tochrome b5 transmembrane sequence chimeras do not inhibit SERCA2a. FEBS Letters, 425, 509-512. doi:10.1016/S0014-5793(98)00151-3 [165] Deborah, A., Ferrington, Q.Y., Thomas, C.S. and Bige- low, D.J. (2002) Comparable Levels of CaATPase Inhi- bition by Phospholamban in Slow-Twitch Skeletal and Cardiac Sarcoplasmic Reticulum. Biochemistry, 41, 13289- 13296. doi:10.1021/bi026407t [166] Uemura, N., Ohkusa, T., Hamano, K., Nakagome, M., Hori, H., Shimizu, M., Matsuzaki, M., Mochizuki, S., Minami- sawa, S. and Ishikawa, Y. (2004) Down-regulation of sar- colipin mRNA expression in chronic atrial fibrillation. Euro- pean Journal of Clinical Investigation, 34, 723-730. doi:10.1111/j.1365-2362.2004.01422.x [167] Odermatt, A., Taschner, P.E., Scherer, S.W., Beatty, B., Khanna, V.K., Cornblath, D.R., Chaudhry, V., Yee, W.C., Schrank, B., Karpati, G., Breuning, M.H., Knoers N. and MacLennan, D.H. (1997) Characterization of the gene en- coding human sarcolipin (SLN), a proteolipid associated with SERCA1: absence of structural mutations in five pa- tients with Brody disease. Genomics, 45, 541-553. doi:10.1006/geno.1997.4967 [168] Asahi, M., Nakayama, H., Tada, M. and Otsu, K. (2003) Regulation of sarco (endo)plasmic reticulum Ca2+ adenosine triphosphatase by phospholamban and sarcolipin: implica- tion for cardiac hypertrophy and failure. Trends in Cardio- vascular Medicine, 13, 152-157. doi:10.1016/S1050-1738(03)00037-9 [169] Mascioni, A., Karim, C., Barany, G., Thomas, D.D. and Veglia, G. (2002) Structure and orientation of sarcolipin in lipid environments. Biochemistry, 41, 475-482. doi:10.1021/bi011243m [170] Buffy, J.J., Buck-Koehntop, B.A., Porcelli, F., Traaseth, N.J., Thomas, D.D. and Veglia, G. (2006) Defining the intramem- brane binding mechanism of sarcolipin to calcium ATPase using solution NMR spectroscopy. Journal of Molecular Bi- ology, 358, 420-429. doi:10.1016/j.jmb.2006.02.005 [171] Gramolini, A.O., Kislinger, T., Asahi, M., Li, W., Emili, A. and MacLennan, D.H. (2004) Sarcolipin retention in th e endoplasmic reticulum depends on its C-terminal RSYQY sequence and its interaction with sarco(endo)plasmic Ca2+- ATPases. Proceedings of the National Academy of Sciences of the USA, 101, 16807-16812. doi:10.1073/pnas.0407815101 [172] Morita, T., Hussain, D., Asahi, M., Tsuda, T., Kurzydlowski, K., Toyoshima, C. and Maclennan, D.H. (2008) Interaction sites among phospholamban, sarcolipin, and the sarco (endo) plasmic reticulum Ca2+-ATPase. Biochemical Biophysical Research Communication, 369, 188-194. doi:10.1016/j.bbrc.2007.11.098
|