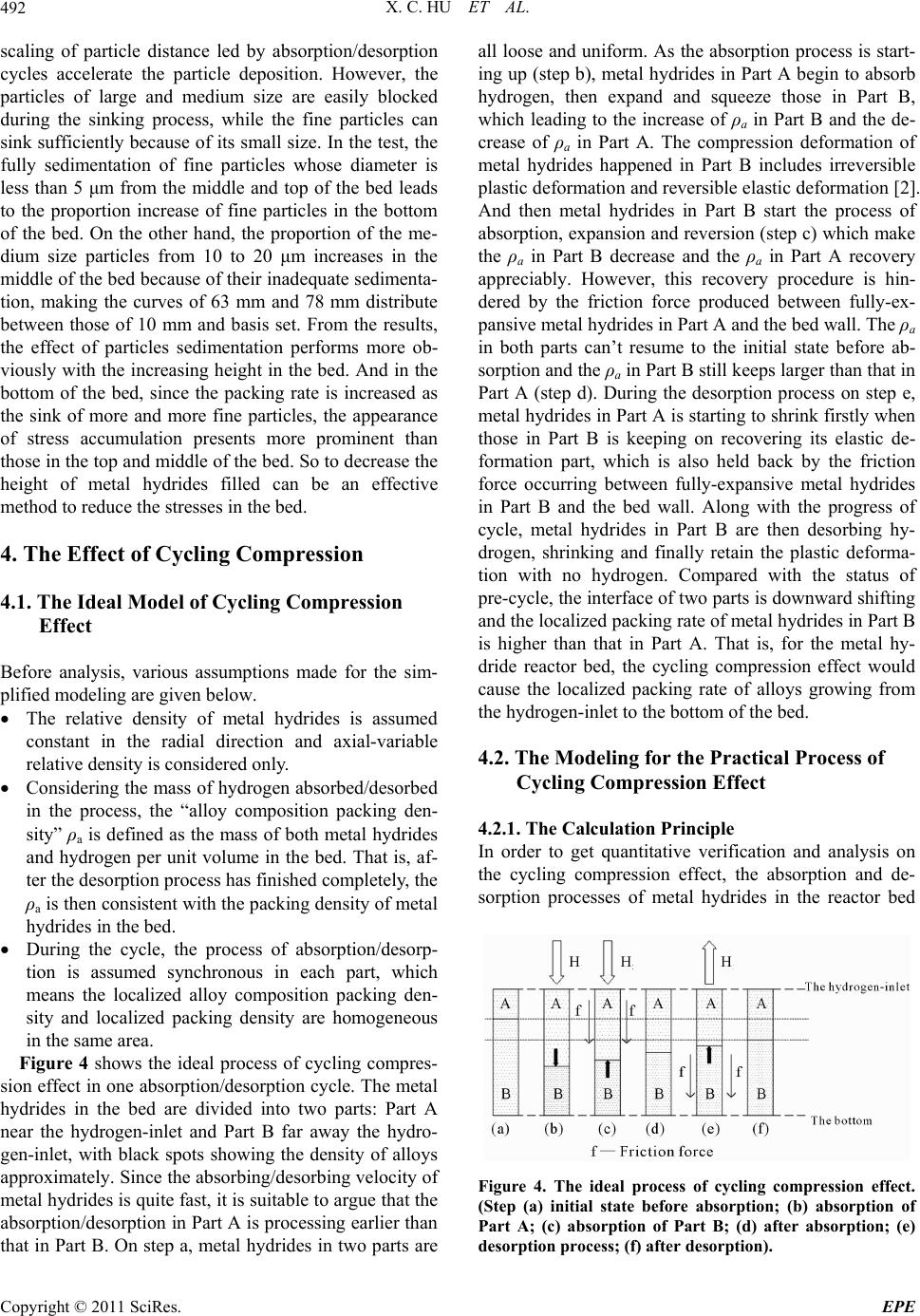
X. C. HU ET AL.
492
scaling of particle distance led by absorption/desorption
cycles accelerate the particle deposition. However, the
particles of large and medium size are easily blocked
during the sinking process, while the fine particles can
sink sufficiently because of its small size. In the test, the
fully sedimentation of fine particles whose diameter is
less than 5 μm from the middle and top of the bed leads
to the proportion increase of fine particles in the bottom
of the bed. On the other hand, the proportion of the me-
dium size particles from 10 to 20 μm increases in the
middle of the bed because of their inadequate sedimenta-
tion, making the curves of 63 mm and 78 mm distribute
between those of 10 mm and basis set. From the results,
the effect of particles sedimentation performs more ob-
viously with the increasing height in the bed. And in the
bottom of the bed, since the packing rate is increased as
the sink of more and more fine particles, the appearance
of stress accumulation presents more prominent than
those in the top and middle of the bed. So to decrease the
height of metal hydrides filled can be an effective
method to reduce the stresses in the bed.
4. The Effect of Cycling Compression
4.1. The Ideal Model of Cycling Compression
Effect
Before analysis, various assumptions made for the sim-
plified modeling are given below.
The relative density of metal hydrides is assumed
constant in the radial direction and axial-variable
relative density is considered only.
Considering the mass of hydrogen absorbed/desorbed
in the process, the “alloy composition packing den-
sity” ρa is defined as the mass of both metal hydrides
and hydrogen per unit volume in the bed. That is, af-
ter the desorption process has finished completely, the
ρa is then consistent with the packing density of metal
hydrides in the bed.
During the cycle, the process of absorption/desorp-
tion is assumed synchronous in each part, which
means the localized alloy composition packing den-
sity and localized packing density are homogeneous
in the same area.
Figure 4 shows the ideal process of cycling compres-
sion effect in one absorption/desorption cycle. The metal
hydrides in the bed are divided into two parts: Part A
near the hydrogen-inlet and Part B far away the hydro-
gen-inlet, with black spots showing the density of alloys
approximately. Since the absorbing/desorbing velocity of
metal hydrides is quite fast, it is suitable to argue that the
absorption/desorption in Part A is processing earlier than
that in Part B. On step a, metal hydrides in two parts are
all loose and uniform. As the absorption process is start-
ing up (step b), metal hydrides in Part A begin to absorb
hydrogen, then expand and squeeze those in Part B,
which leading to the increase of ρa in Part B and the de-
crease of ρa in Part A. The compression deformation of
metal hydrides happened in Part B includes irreversible
plastic deformation and reversible elastic deformation [2].
And then metal hydrides in Part B start the process of
absorption, expansion and reversion (step c) which make
the ρa in Part B decrease and the ρa in Part A recovery
appreciably. However, this recovery procedure is hin-
dered by the friction force produced between fully-ex-
pansive metal hydrides in Part A and the bed wall. The ρa
in both parts can’t resume to the initial state before ab-
sorption and the ρa in Part B still keeps larger than that in
Part A (step d). During the desorption process on step e,
metal hydrides in Part A is starting to shrink firstly when
those in Part B is keeping on recovering its elastic de-
formation part, which is also held back by the friction
force occurring between fully-expansive metal hydrides
in Part B and the bed wall. Along with the progress of
cycle, metal hydrides in Part B are then desorbing hy-
drogen, shrinking and finally retain the plastic deforma-
tion with no hydrogen. Compared with the status of
pre-cycle, the interface of two parts is downward shifting
and the localized packing rate of metal hydrides in Part B
is higher than that in Part A. That is, for the metal hy-
dride reactor bed, the cycling compression effect would
cause the localized packing rate of alloys growing from
the hydrogen-inlet to the bottom of the bed.
4.2. The Modeling for the Practical Process of
Cycling Compression Effect
4.2.1. The Calculation Principle
In order to get quantitative verification and analysis on
the cycling compression effect, the absorption and de-
sorption processes of metal hydrides in the reactor bed
Figure 4. The ideal process of cycling compression effect.
(Step (a) initial state before absorption; (b) absorption of
Part A; (c) absorption of Part B; (d) after absorption; (e)
desorption process; (f) after desorption).
Copyright © 2011 SciRes. EPE