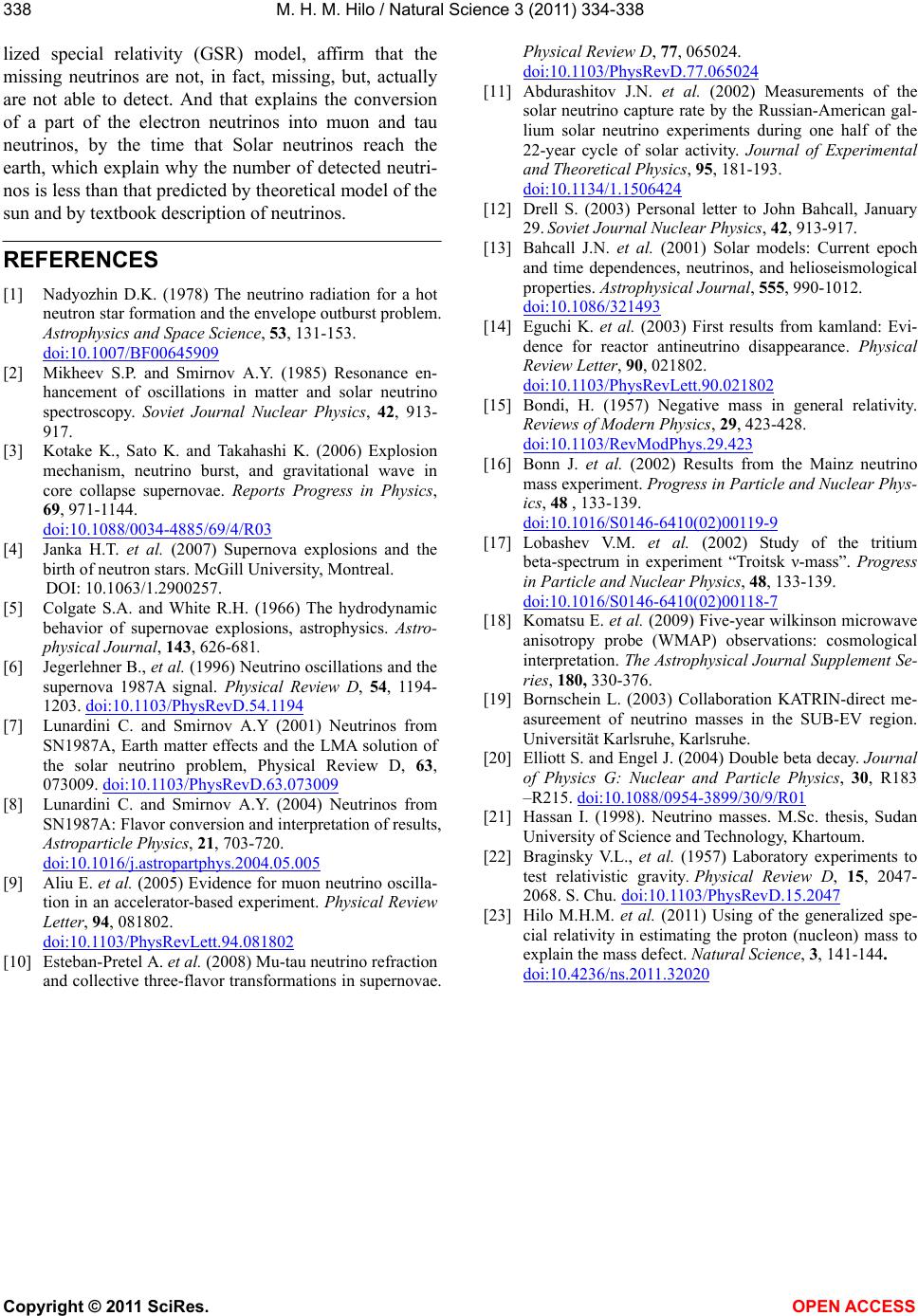
M. H. M. Hilo / Natural Science 3 (2011) 334-338
Copyright © 2011 SciRes. OPEN ACCESS
338
lized special relativity (GSR) model, affirm that the
missing neutrinos are not, in fact, missing, but, actually
are not able to detect. And that explains the conversion
of a part of the electron neutrinos into muon and tau
neutrinos, by the time that Solar neutrinos reach the
earth, which explain why the number of detected neutri-
nos is less than that predicted by theoretical model of the
sun and by textbook description of neutrinos.
REFERENCES
[1] Nadyozhin D.K. (1978) The neutrino radiation for a hot
neutron star formation and the envelope outburst problem.
Astrophysics and Space Science, 53, 131-153.
doi:10.1007/BF00645909
[2] Mikheev S.P. and Smirnov A.Y. (1985) Resonance en-
hancement of oscillations in matter and solar neutrino
spectroscopy. Soviet Journal Nuclear Physics, 42, 913-
917.
[3] Kotake K., Sato K. and Takahashi K. (2006) Explosion
mechanism, neutrino burst, and gravitational wave in
core collapse supernovae. Reports Progress in Physics,
69, 971-1144.
doi:10.1088/0034-4885/69/4/R03
[4] Janka H.T. et al. (2007) Supernova explosions and the
birth of neutron stars. McGill University, Montreal.
DOI: 10.1063/1.2900257.
[5] Colgate S.A. and White R.H. (1966) The hydrodynamic
behavior of supernovae explosions, astrophysics. Astro-
physical Journal, 143, 626-681.
[6] Jegerlehner B., et al. (1996) Neutrino oscillations and the
supernova 1987A signal. Physical Review D, 54, 1194-
1203. doi:10.1103/PhysRevD.54.1194
[7] Lunardini C. and Smirnov A.Y (2001) Neutrinos from
SN1987A, Earth matter effects and the LMA solution of
the solar neutrino problem, Physical Review D, 63,
073009. doi:10.1103/PhysRevD.63.073009
[8] Lunardini C. and Smirnov A.Y. (2004) Neutrinos from
SN1987A: Flavor conversion and interpretation of results,
Astroparticle Physics, 21, 703-720.
doi:10.1016/j.astropartphys.2004.05.005
[9] Aliu E. et al. (2005) Evidence for muon neutrino oscilla-
tion in an accelerator-based experiment. Physical Review
Letter, 94, 081802.
doi:10.1103/PhysRevLett.94.081802
[10] Esteban-Pretel A. et al. (2008) Mu-tau neutrino refraction
and collective three-flavor transformations in supernovae.
Physical Review D, 77, 065024.
doi:10.1103/PhysRevD.77.065024
[11] Abdurashitov J.N. et al. (2002) Measurements of the
solar neutrino capture rate by the Russian-American gal-
lium solar neutrino experiments during one half of the
22-year cycle of solar activity. Journal of Experimental
and Theoretical Physics, 95, 181-193.
doi:10.1134/1.1506424
[12] Drell S. (2003) Personal letter to John Bahcall, January
29. Soviet Journal Nuclear Physics, 42, 913-917.
[13] Bahcall J.N. et al. (2001) Solar models: Current epoch
and time dependences, neutrinos, and helioseismological
properties. Astrophysical Journal, 555, 990-1012.
doi:10.1086/321493
[14] Eguchi K. et al. (2003) First results from kamland: Evi-
dence for reactor antineutrino disappearance. Physical
Review Letter, 90, 021802.
doi:10.1103/PhysRevLett.90.021802
[15] Bondi, H. (1957) Negative mass in general relativity.
Reviews of Modern Physics, 29, 423-428.
doi:10.1103/RevModPhys.29.423
[16] Bonn J. et al. (2002) Results from the Mainz neutrino
mass experiment. Progress in Particle and Nuclear Phys-
ics, 48 , 133-139.
doi:10.1016/S0146-6410(02)00119-9
[17] Lobashev V.M. et al. (2002) Study of the tritium
beta-spectrum in experiment “Troitsk ν-mass”. Progress
in Particle and Nuclear Physics, 48, 133-139.
doi:10.1016/S0146-6410(02)00118-7
[18] Komatsu E. et al. (2009) Five-year wilkinson microwave
anisotropy probe (WMAP) observations: cosmological
interpretation. The Astrophysical Journal Supplement Se-
ries, 180, 330-376.
[19] Bornschein L. (2003) Collaboration KATRIN-direct me-
asureement of neutrino masses in the SUB-EV region.
Universität Karlsruhe, Karlsruhe.
[20] Elliott S. and Engel J. (2004) Double beta decay. Journal
of Physics G: Nuclear and Particle Physics, 30, R183
–R215. doi:10.1088/0954-3899/30/9/R01
[21] Hassan I. (1998). Neutrino masses. M.Sc. thesis, Sudan
University of Science and Technology, Khartoum.
[22] Braginsky V.L., et al. (1957) Laboratory experiments to
test relativistic gravity. Physical Review D, 15, 2047-
2068. S. Chu. doi:10.1103/PhysRevD.15.2047
[23] Hilo M.H.M. et al. (2011) Using of the generalized spe-
cial relativity in estimating the proton (nucleon) mass to
explain the mass defect. Natural Science, 3, 141-144.
doi:10.4236/ns.2011.32020