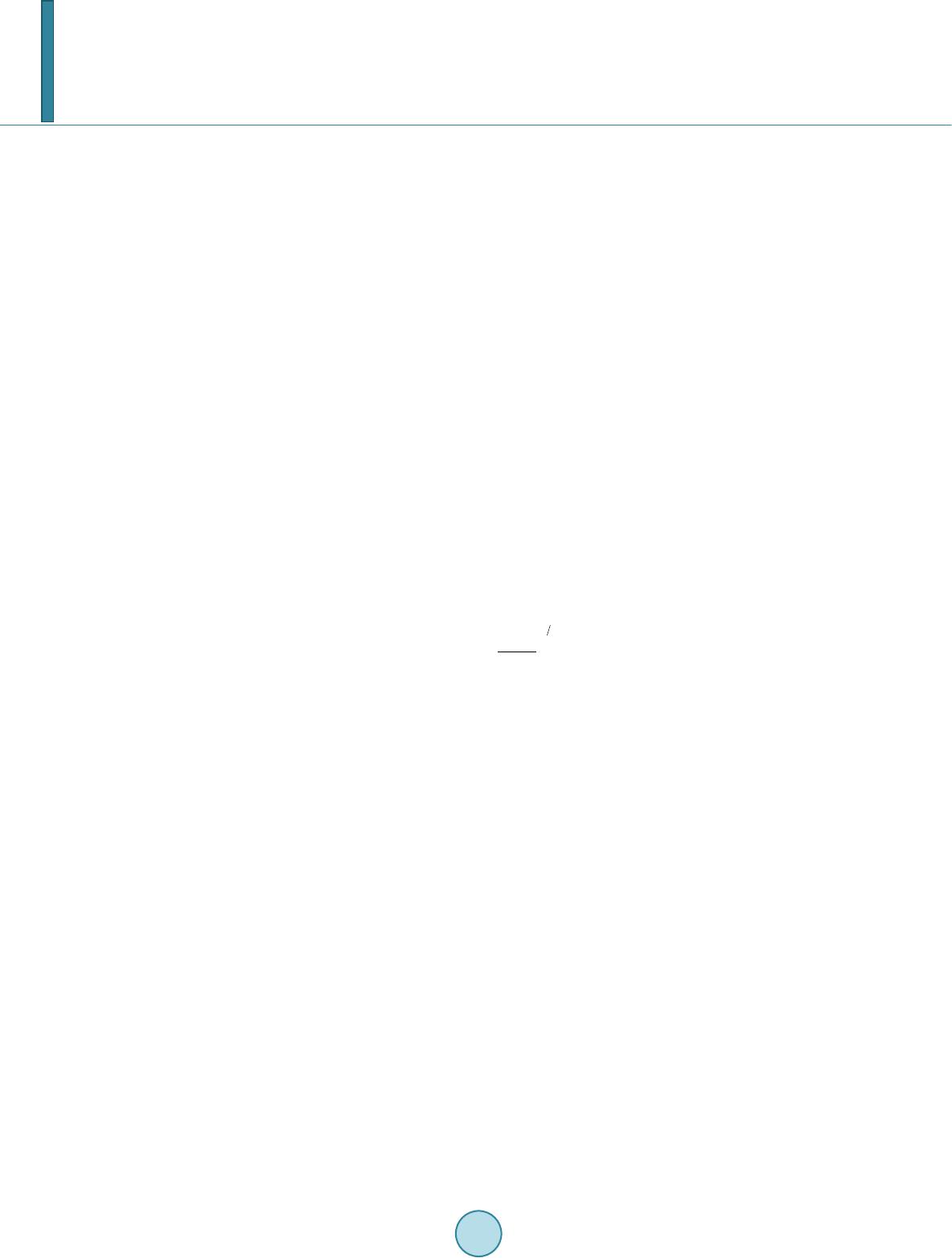
A. Adebayo, M. Mahmoud
ture and sequestration (CCS) must be at this depth (Benson, 2008). While CO2 is injected into a subsurface for-
mation, four major trapping mechanisms ensue namely: 1) Physical/Structural trapping—a process whereby
impermeable cap rock at the top of the formation prevent the CO2 from escaping out of the formation, keeping it
in place (Benson, 2008; W ang, 2010), 2) Solubility trapping—a process whereby injected CO2 dissolves in for-
mation pore water, 3) Mineral trapping—occurs when aqueous CO2 reacts with formation rock minerals pro-
ducing precipitates of carbonate minerals, and 4) Residual or Capillary trapping which occurs after CO2 injec-
tion stops and water begins to imbibe into the aquifer displacing the CO2 already in the aquifer. Not all the CO2
is displaced but some are left behind as residual CO2 (residual trapping). Dissolved CO2 can acidify formation
water and subsequently mobilize and transport trace metals as it migrates in the formation (Gaus, 2010). A stor-
age formation with strong cap rock is thus crucial to avoid leakages of trace metals and compounds into overly-
ing portable aquifers. There is also the need for proper monitoring of CO2 migration so as to observe its behav-
ior, distribution, and possible leakages. Hence, evaluation of the reliability of CO2 monitoring techniques should
be first step in risk assessment of any CCS project (Wang, 2004). Techniques required for monitoring CO2 mi-
gration can be borrowed from a variety of other applications such as those used in the oil and gas industry, and
those used in ground water monitoring (Benson, 2008). Examples of such techniques are seismic, gravity, and
resistivity measurements. Seismic is the most extensively used technique. The use of resistivity measurements is
also promising. Resistivity technique is applicable because rocks contain saline water and are thus conductive.
The use of resistivity measurements to monitor and quantify CO2 in underground geological formation depends
on the fact that formation resistivity is higher at locations occupied by CO2 compared to locations un-invaded by
CO2, and the resistivity increases as CO2 saturation increases. The amount of resistivity changes depend on the
volume of CO2 present. With the use of Archie’s equation or modified Archie’s equation, CO2 saturation distri-
bution, migration, and volume can be estimated. Many experimental and field studies have successfully used re-
sistivity measurements to monitor CO2 migration (Ramire z, 2003; Wa ng, 2010; Gie se, 200 9; Chr iste nse n, 2006;
Nakat su ka, 2010). Archie’s equation is given in Equation (1).
(1)
where “a” is a constant determined as the intercept passing through 100% porosity on a formation fac-
tor-porosity plot, while “m” and “n” are cementation factor and saturation exponent respectively and are ob-
tained from laboratory resistivity measurements on core samples.
Kiessling et al. (2010) applied Archie’s equation to estimate CO2 saturation distribution in a CO2 SINK test
site close to Ketzin (Germany). Similarly, Nakatsuka et al. (2010) reported the use of Archie and modified
Archie equation to estimate CO2 distribution in the Nagaoka pilot CO2 injection site. Time lapse resistivity
measurements in both cases were used to monitor movement of CO2 to and away from different zones of interest.
Changing resistivity values were interpreted as change in CO2 saturation. Figure 1 shows the time lapse resis-
tivity logs for both cases.
This study is new compared to previous works in the following sense: Many previous laboratory works have fo-
cused on the applicability of electrical resistivity measurements to track CO2 migration by way of resistivity change
as a function of CO2 saturation changes during CO2 sequestration. Such experiments were also conducted only
within several hours or less. The fate of formation resistivity in the event that the CO2 remained trapped in the
pores for an extended period of time and the subsequent effect of the CO2/brine/rock reactions on resistivity and on
CO2 monitoring and estimation has not been addressed. This paper investigates the effect of CO2 brine rock reac-
tion on resistivity and CO2 saturation estimation.
2. Materials and Methods
All samples were collected from the same quarry rock of Indiana lime stone with recorded high level of homo-
geneity. Cylindrical samples with dimension of 3.74 cm diameter and length of 7 cm were cut and surface
grinded to ensure very flat end faces. Samples were cleaned by solvent reflux method and vacuum dried in oven
at 80˚C. Sample properties were then measured and tabulated in Table 1. De-aerated synthetic brine with com-
position and properties shown in Table 2 wer e prepared and used to saturate core samples.