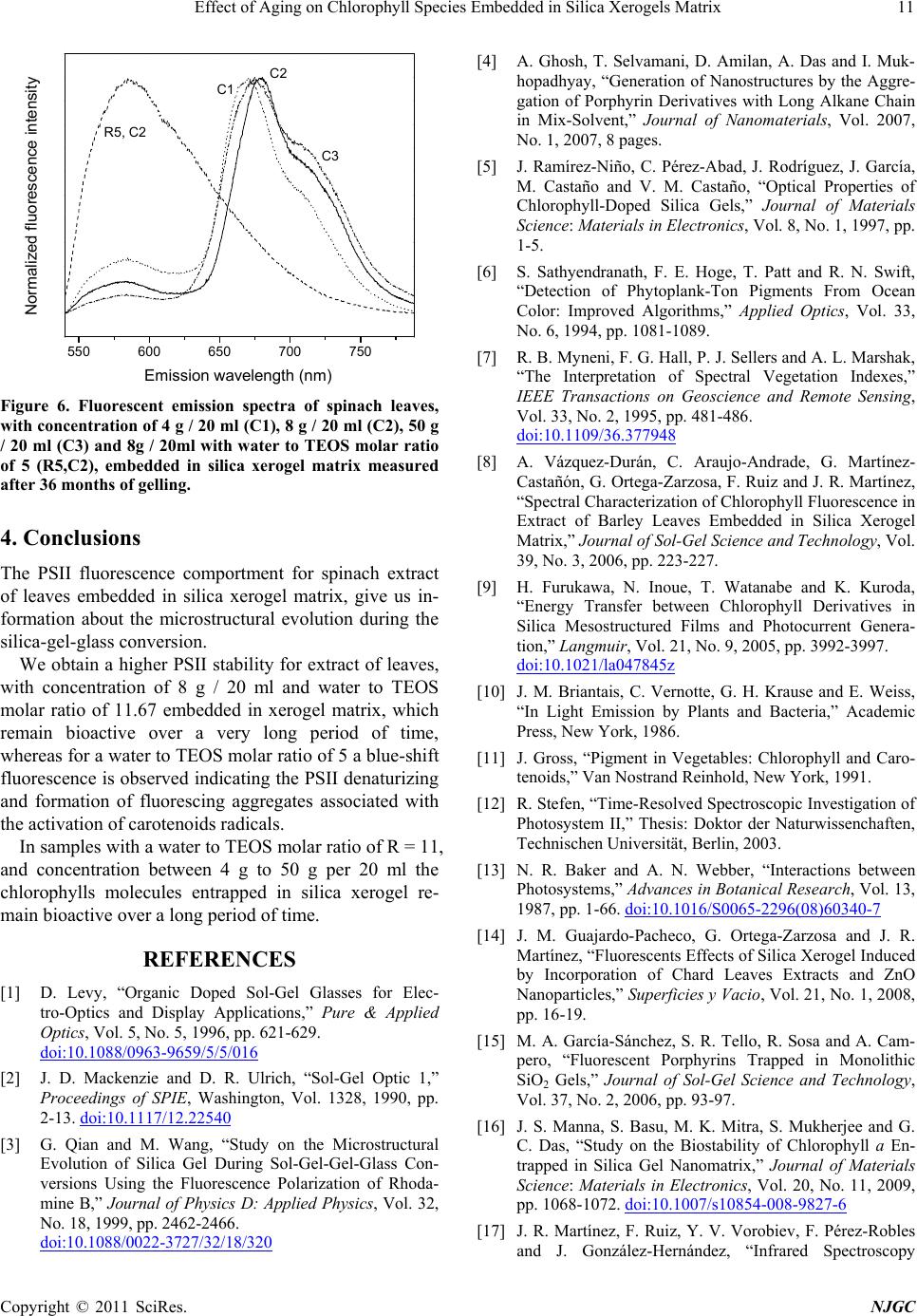
Effect of Aging on Chlorophyll Species Embedded in Silica Xerogels Matrix11
550 600650 700750
Normalized fluorescence intensity
Emission wavelength (nm)
R5, C2
C2
C1
C3
Figure 6. Fluorescent emission spectra of spinach leaves,
with concentration of 4 g / 20 ml (C1), 8 g / 20 ml (C2), 50 g
/ 20 ml (C3) and 8g / 20ml with water to TEOS molar ratio
of 5 (R5,C2), embedded in silica xerogel matrix measured
after 36 months of gelling.
4. Conclusions
The PSII fluorescence comportment for spinach extract
of leaves embedded in silica xerogel matrix, give us in-
formation about the microstructural evolution during the
silica-gel-glass conversion.
We obtain a higher PSII stability for extract of leaves,
with concentration of 8 g / 20 ml and water to TEOS
molar ratio of 11.67 embedded in xerogel matrix, which
remain bioactive over a very long period of time,
whereas for a water to TEOS molar ratio of 5 a blue-shift
fluorescence is observed indicating the PSII denaturizing
and formation of fluorescing aggregates associated with
the activation of carotenoids radical s.
In samples with a water to TEOS molar ratio of R = 11,
and concentration between 4 g to 50 g per 20 ml the
chlorophylls molecules entrapped in silica xerogel re-
main bioactive ov er a long period of time.
REFERENCES
[1] D. Levy, “Organic Doped Sol-Gel Glasses for Elec-
tro-Optics and Display Applications,” Pure & Applied
Optics, Vol. 5, No. 5, 1996, pp. 621-629.
doi:10.1088/0963-9659/5/5/016
[2] J. D. Mackenzie and D. R. Ulrich, “Sol-Gel Optic 1,”
Proceedings of SPIE, Washington, Vol. 1328, 1990, pp.
2-13. doi:10.1117/12.22540
[3] G. Qian and M. Wang, “Study on the Microstructural
Evolution of Silica Gel During Sol-Gel-Gel-Glass Con-
versions Using the Fluorescence Polarization of Rhoda-
mine B,” Journal of Physics D: Applied Physics, Vol. 32,
No. 18, 1999, pp. 2462-2466.
doi:10.1088/0022-3727/32/18/320
[4] A. Ghosh, T. Selvamani, D. Amilan, A. Das and I. Muk-
hopadhyay, “Generation of Nanostructures by the Aggre-
gation of Porphyrin Derivatives with Long Alkane Chain
in Mix-Solvent,” Journal of Nanomaterials, Vol. 2007,
No. 1, 2007, 8 pages.
[5] J. Ramírez-Niño, C. Pérez-Abad, J. Rodríguez, J. García,
M. Castaño and V. M. Castaño, “Optical Properties of
Chlorophyll-Doped Silica Gels,” Journal of Materials
Science: Materials in Electronics, Vol. 8, No. 1, 1997, pp.
1-5.
[6] S. Sathyendranath, F. E. Hoge, T. Patt and R. N. Swift,
“Detection of Phytoplank-Ton Pigments From Ocean
Color: Improved Algorithms,” Applied Optics, Vol. 33,
No. 6, 1994, pp. 1081-1089.
[7] R. B. Myneni, F. G. Hall, P. J. Sellers and A. L. Marshak,
“The Interpretation of Spectral Vegetation Indexes,”
IEEE Transactions on Geoscience and Remote Sensing,
Vol. 33, No. 2, 1995, pp. 481-486.
doi:10.1109/36.377948
[8] A. Vázquez-Durán, C. Araujo-Andrade, G. Martínez-
Castañón, G. Ortega-Zarzosa, F. Ruiz and J. R. Martínez,
“Spectral Characterization of Chlorophyll Fluorescence in
Extract of Barley Leaves Embedded in Silica Xerogel
Matrix,” Journal of Sol-Gel Science and Technology, Vol.
39, No. 3, 2006, pp. 223-227.
[9] H. Furukawa, N. Inoue, T. Watanabe and K. Kuroda,
“Energy Transfer between Chlorophyll Derivatives in
Silica Mesostructured Films and Photocurrent Genera-
tion,” Langmuir, Vol. 21, No. 9, 2005, pp. 3992-3997.
doi:10.1021/la047845z
[10] J. M. Briantais, C. Vernotte, G. H. Krause and E. Weiss,
“In Light Emission by Plants and Bacteria,” Academic
Press, New York, 1986.
[11] J. Gross, “Pigment in Vegetables: Chlorophyll and Caro-
tenoids,” Van Nostrand Reinhold, New York, 1991.
[12] R. Stefen, “Time-Resolved Spectroscopic Investigation of
Photosystem II,” Thesis: Doktor der Naturwissenchaften,
Technischen Universität, Berlin, 2003.
[13] N. R. Baker and A. N. Webber, “Interactions between
Photosystems,” Advances in Botanical Research, Vol. 13,
1987, pp. 1-66. doi:10.1016/S0065-2296(08)60340-7
[14] J. M. Guajardo-Pacheco, G. Ortega-Zarzosa and J. R.
Martínez, “Fluorescents Effects of Silica Xerogel Induced
by Incorporation of Chard Leaves Extracts and ZnO
Nanoparticles,” Superficies y Vacio, Vol. 21, No. 1, 2008,
pp. 16-19.
[15] M. A. García-Sánchez, S. R. Tello, R. Sosa and A. Cam-
pero, “Fluorescent Porphyrins Trapped in Monolithic
SiO2 Gels,” Journal of Sol-Gel Science and Technology,
Vol. 37, No. 2, 2006, pp. 93-97.
[16] J. S. Manna, S. Basu, M. K. Mitra, S. Mukherjee and G.
C. Das, “Study on the Biostability of Chlorophyll a En-
trapped in Silica Gel Nanomatrix,” Journal of Materials
Science: Materials in Electronics, Vol. 20, No. 11, 2009,
pp. 1068-1072. doi:10.1007/s10854-008-9827-6
[17] J. R. Martínez, F. Ruiz, Y. V. Vorobiev, F. Pérez-Robles
and J. González-Hernández, “Infrared Spectroscopy
Copyright © 2011 SciRes. NJGC