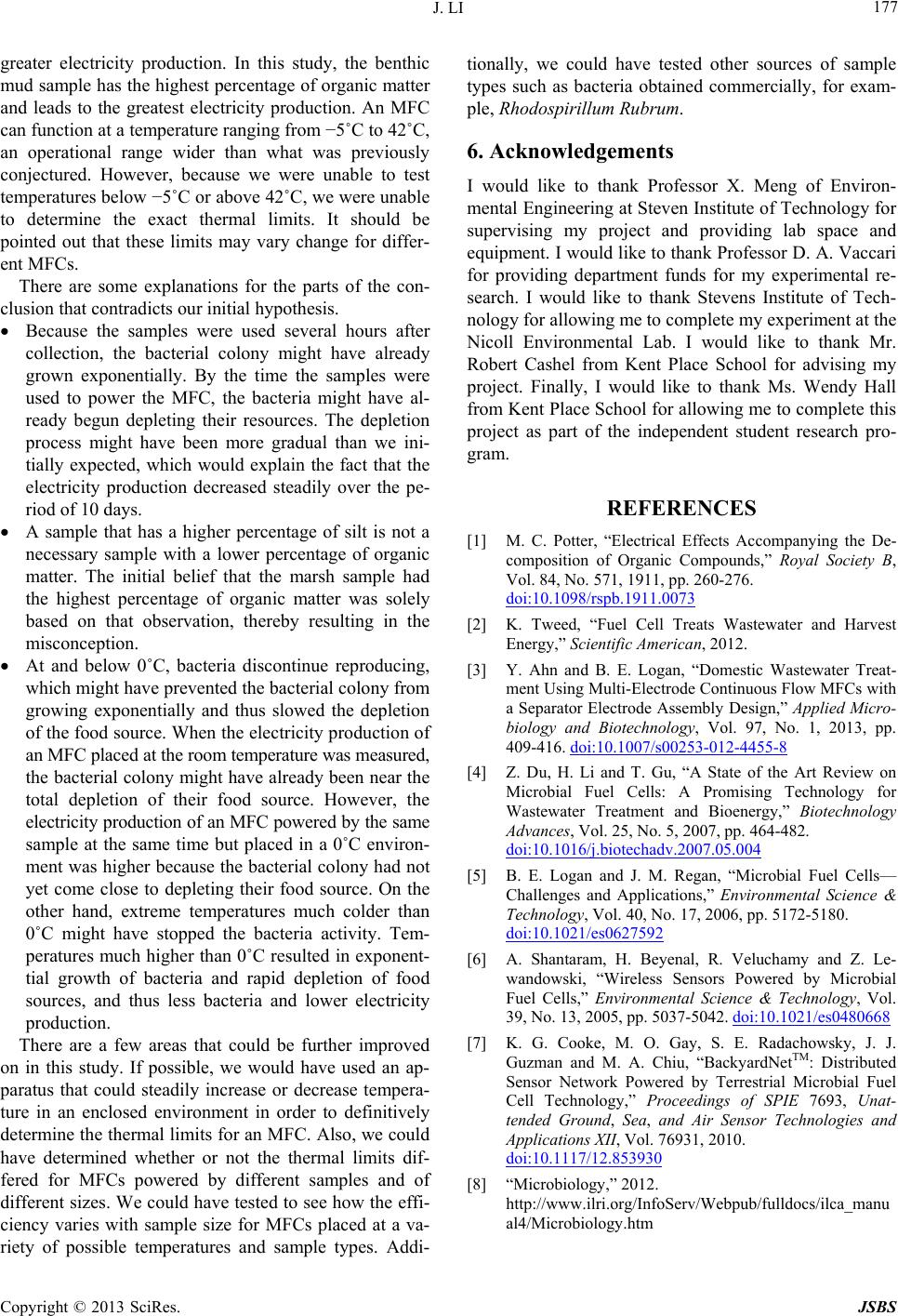
J. LI
Copyright © 2013 SciRes. JSBS
177
greater electricity production. In this study, the benthic
mud sample has the highest percentage of organic matter
and leads to the greatest electricity production. An MFC
can function at a temperature ranging from −5˚C to 42˚C,
an operational range wider than what was previously
conjectured. However, because we were unable to test
temperatures below −5˚C or above 42˚C, we were unable
to determine the exact thermal limits. It should be
pointed out that these limits may vary change for differ-
ent MFCs.
There are some explanations for the parts of the con-
clusion that contradicts our initial hypothesis.
Because the samples were used several hours after
collection, the bacterial colony might have already
grown exponentially. By the time the samples were
used to power the MFC, the bacteria might have al-
ready begun depleting their resources. The depletion
process might have been more gradual than we ini-
tially expected, which would explain the fact that the
electricity production decreased steadily over the pe-
riod of 10 days.
A sample that has a higher percentage of silt is not a
necessary sample with a lower percentage of organic
matter. The initial belief that the marsh sample had
the highest percentage of organic matter was solely
based on that observation, thereby resulting in the
misconception.
At and below 0˚C, bacteria discontinue reproducing,
which might have prevented the bacterial colony from
growing exponentially and thus slowed the depletion
of the food source. When the electricity production of
an MFC placed at the room temperature was measured,
the bacterial colony might have already been near the
total depletion of their food source. However, the
electricity production of an MFC powered by the same
sample at the same time but placed in a 0˚C environ-
ment was higher because the bacterial colony had not
yet come close to depleting their food source. On the
other hand, extreme temperatures much colder than
0˚C might have stopped the bacteria activity. Tem-
peratures much higher than 0˚C resulted in exponent-
tial growth of bacteria and rapid depletion of food
sources, and thus less bacteria and lower electricity
production.
There are a few areas that could be further improved
on in this study. If possible, we would have used an ap-
paratus that could steadily increase or decrease tempera-
ture in an enclosed environment in order to definitively
determine the thermal limits for an MFC. Also, we could
have determined whether or not the thermal limits dif-
fered for MFCs powered by different samples and of
different sizes. We could have tested to see how the effi-
ciency varies with sample size for MFCs placed at a va-
riety of possible temperatures and sample types. Addi-
tionally, we could have tested other sources of sample
types such as bacteria obtained commercially, for exam-
ple, Rhodospirillum Rubrum.
6. Acknowledgements
I would like to thank Professor X. Meng of Environ-
mental Engineering at Steven Institute of Technology for
supervising my project and providing lab space and
equipment. I would like to thank Professor D. A. Vaccari
for providing department funds for my experimental re-
search. I would like to thank Stevens Institute of Tech-
nology for allowing me to complete my experiment at the
Nicoll Environmental Lab. I would like to thank Mr.
Robert Cashel from Kent Place School for advising my
project. Finally, I would like to thank Ms. Wendy Hall
from Kent Place School for allowing me to complete this
project as part of the independent student research pro-
gram.
REFERENCES
[1] M. C. Potter, “Electrical Effects Accompanying the De-
composition of Organic Compounds,” Royal Society B,
Vol. 84, No. 571, 1911, pp. 260-276.
doi:10.1098/rspb.1911.0073
[2] K. Tweed, “Fuel Cell Treats Wastewater and Harvest
Energy,” Scientific American, 2012.
[3] Y. Ahn and B. E. Logan, “Domestic Wastewater Treat-
ment Using Multi-Electrode Continuous Flow MFCs with
a Separator Electrode Assembly Design,” Applied Micro-
biology and Biotechnology, Vol. 97, No. 1, 2013, pp.
409-416. doi:10.1007/s00253-012-4455-8
[4] Z. Du, H. Li and T. Gu, “A State of the Art Review on
Microbial Fuel Cells: A Promising Technology for
Wastewater Treatment and Bioenergy,” Biotechnology
Advances, Vol. 25, No. 5, 2007, pp. 464-482.
doi:10.1016/j.biotechadv.2007.05.004
[5] B. E. Logan and J. M. Regan, “Microbial Fuel Cells—
Challenges and Applications,” Environmental Science &
Technology, Vol. 40, No. 17, 2006, pp. 5172-5180.
doi:10.1021/es0627592
[6] A. Shantaram, H. Beyenal, R. Veluchamy and Z. Le-
wandowski, “Wireless Sensors Powered by Microbial
Fuel Cells,” Environmental Science & Technology, Vol.
39, No. 13, 2005, pp. 5037-5042. doi:10.1021/es0480668
[7] K. G. Cooke, M. O. Gay, S. E. Radachowsky, J. J.
Guzman and M. A. Chiu, “BackyardNetTM: Distributed
Sensor Network Powered by Terrestrial Microbial Fuel
Cell Technology,” Proceedings of SPIE 7693, Unat-
tended Ground, Sea, and Air Sensor Technologies and
Applications XII, Vol. 76931, 2010.
doi:10.1117/12.853930
[8] “Microbiology,” 2012.
http://www.ilri.org/InfoServ/Webpub/fulldocs/ilca_manu
al4/Microbiology.htm