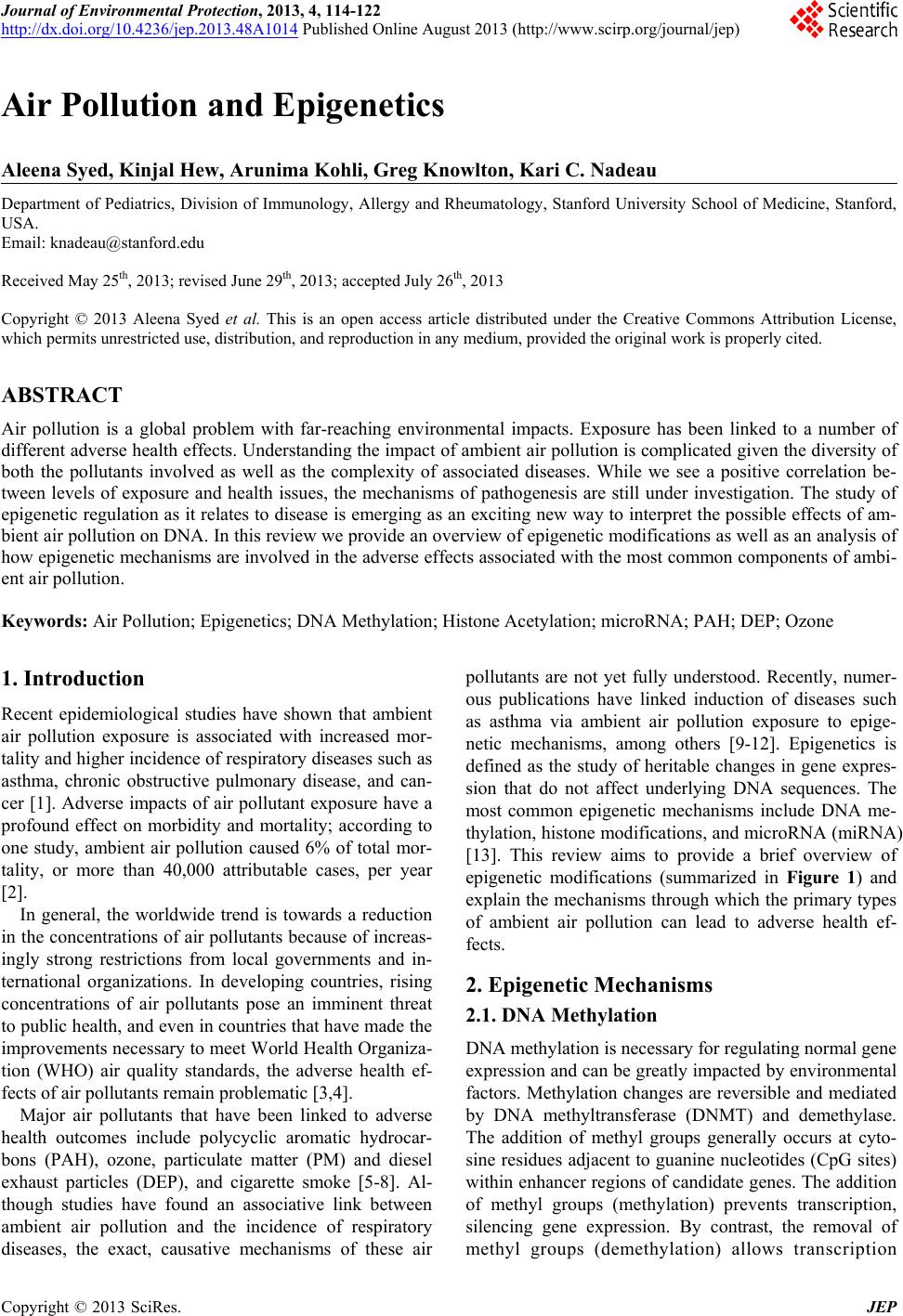 Journal of Environmental Protection, 2013, 4, 114-122 http://dx.doi.org/10.4236/jep.2013.48A1014 Published Online August 2013 (http://www.scirp.org/journal/jep) Air Pollution and Epigenetics Aleena Syed, Kinjal Hew, Arunima Kohli, Greg Knowlton, Kari C. Nadeau Department of Pediatrics, Division of Immunology, Allergy and Rheumatology, Stanford University School of Medicine, Stanford, USA. Email: knadeau@stanford.edu Received May 25th, 2013; revised June 29th, 2013; accepted July 26th, 2013 Copyright © 2013 Aleena Syed et al. This is an open access article distributed under the Creative Commons Attribution License, which permits unrestricted use, distribution, and reproduction in any medium, provided the original work is properly cited. ABSTRACT Air pollution is a global problem with far-reaching environmental impacts. Exposure has been linked to a number of different adverse health effects. Understanding the impact of ambient air pollution is complicated given the diversity of both the pollutants involved as well as the complexity of associated diseases. While we see a positive correlation be- tween levels of exposure and health issues, the mechanisms of pathogenesis are still under investigation. The study of epigenetic regulation as it relates to disease is emerging as an exciting new way to interpret the possible effects of am- bient air pollution on DNA. In this review we provide an overview of epigenetic modifications as well as an analysis of how epigenetic mechanisms are involved in the adverse effects associated with the most common components of ambi- ent air pollution. Keywords: Air Pollution; Epigenetics; DNA Methylation; Histone Acetylation; microRNA; PAH; DEP; Ozone 1. Introduction Recent epidemiological studies have shown that ambient air pollution exposure is associated with increased mor- tality and higher incidence of respiratory diseases such as asthma, chronic obstructive pulmonary disease, and can- cer [1]. Adverse impacts of air pollutant exposure have a profound effect on morbidity and mortality; according to one study, ambient air pollution caused 6% of total mor- tality, or more than 40,000 attributable cases, per year [2]. In general, the worldwide trend is towards a reduction in the concentrations of air pollutants because of increas- ingly strong restrictions from local governments and in- ternational organizations. In developing countries, rising concentrations of air pollutants pose an imminent threat to public health, and even in countries that have made the improvements necessary to meet World Health Organiza- tion (WHO) air quality standards, the adverse health ef- fects of air pollutants remain problematic [3,4]. Major air pollutants that have been linked to adverse health outcomes include polycyclic aromatic hydrocar- bons (PAH), ozone, particulate matter (PM) and diesel exhaust particles (DEP), and cigarette smoke [5-8]. Al- though studies have found an associative link between ambient air pollution and the incidence of respiratory diseases, the exact, causative mechanisms of these air pollutants are not yet fully understood. Recently, numer- ous publications have linked induction of diseases such as asthma via ambient air pollution exposure to epige- netic mechanisms, among others [9-12]. Epigenetics is defined as the study of heritable changes in gene expres- sion that do not affect underlying DNA sequences. The most common epigenetic mechanisms include DNA me- thylation, histone modifications, and microRNA (miRNA) [13]. This review aims to provide a brief overview of epigenetic modifications (summarized in Figure 1) and explain the mechanisms through which the primary types of ambient air pollution can lead to adverse health ef- fects. 2. Epigenetic Mechanisms 2.1. DNA Methylation DNA methylation is necessary for regulating normal gene expression and can be greatly impacted by environmental factors. Methylation changes are reversible and mediated by DNA methyltransferase (DNMT) and demethylase. The addition of methyl groups generally occurs at cyto- sine residues adjacent to guanine nucleotides (CpG sites) within enhancer regions of candidate genes. The addition of methyl groups (methylation) prevents transcription, silencing gene expression. By contrast, the removal of methyl groups (demethylation) allows transcription Copyright © 2013 SciRes. JEP
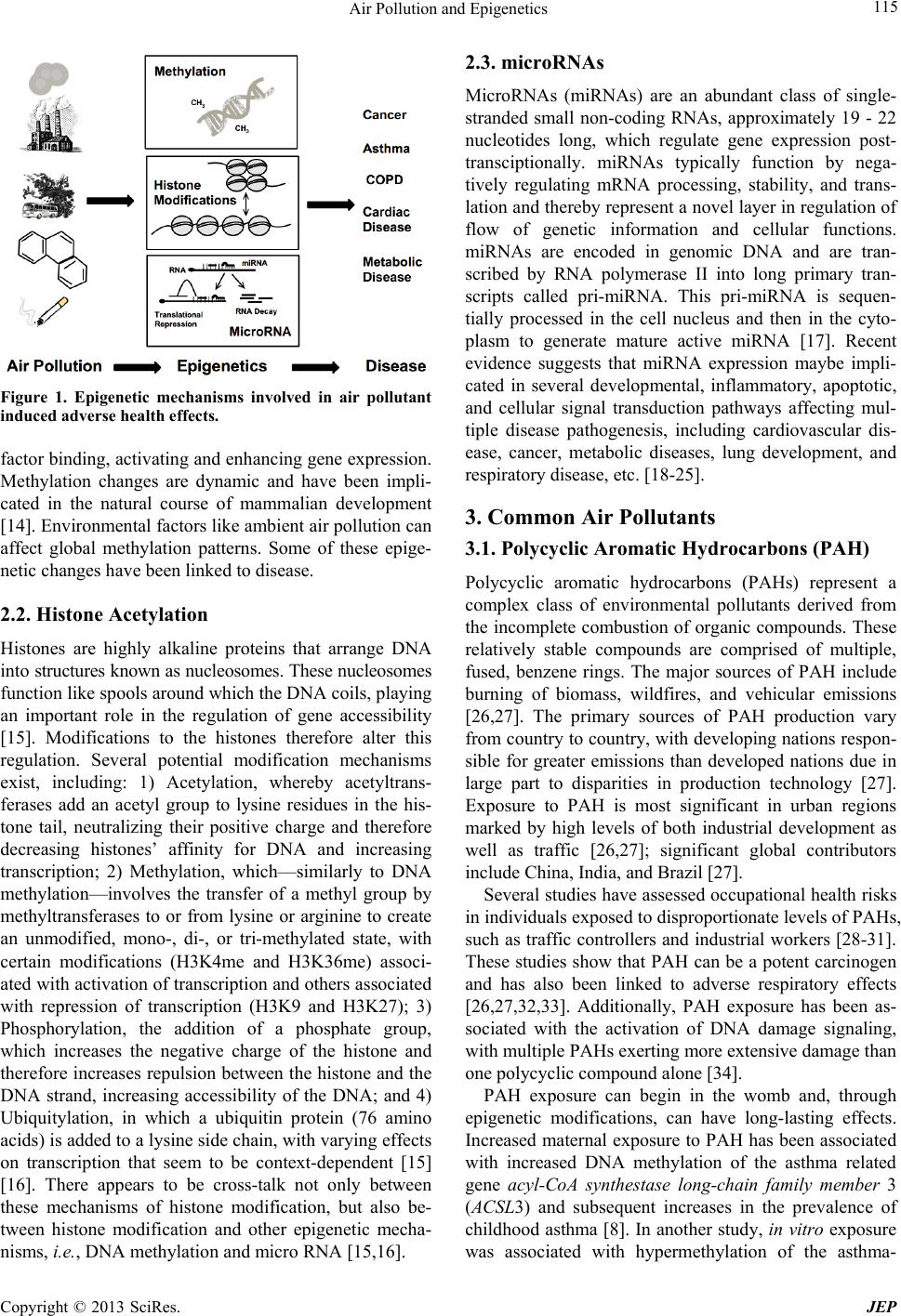 Air Pollution and Epigenetics 115 Figure 1. Epigenetic mechanisms involved in air pollutant induced adverse health effects. factor binding, activating and enhancing gene expression. Methylation changes are dynamic and have been impli- cated in the natural course of mammalian development [14]. Environmental factors like ambient air pollution can affect global methylation patterns. Some of these epige- netic changes have been linked to disease. 2.2. Histone Acetylation Histones are highly alkaline proteins that arrange DNA into structures known as nucleosomes. These nucleosomes function like spools around which the DNA coils, playing an important role in the regulation of gene accessibility [15]. Modifications to the histones therefore alter this regulation. Several potential modification mechanisms exist, including: 1) Acetylation, whereby acetyltrans- ferases add an acetyl group to lysine residues in the his- tone tail, neutralizing their positive charge and therefore decreasing histones’ affinity for DNA and increasing transcription; 2) Methylation, which—similarly to DNA methylation—involves the transfer of a methyl group by methyltransferases to or from lysine or arginine to create an unmodified, mono-, di-, or tri-methylated state, with certain modifications (H3K4me and H3K36me) associ- ated with activation of transcription and others associated with repression of transcription (H3K9 and H3K27); 3) Phosphorylation, the addition of a phosphate group, which increases the negative charge of the histone and therefore increases repulsion between the histone and the DNA strand, increasing accessibility of the DNA; and 4) Ubiquitylation, in which a ubiquitin protein (76 amino acids) is added to a lysine side chain, with varying effects on transcription that seem to be context-dependent [15] [16]. There appears to be cross-talk not only between these mechanisms of histone modification, but also be- tween histone modification and other epigenetic mecha- nisms, i.e., DNA methylation and micro RNA [15,16]. 2.3. microRNAs MicroRNAs (miRNAs) are an abundant class of single- stranded small non-coding RNAs, approximately 19 - 22 nucleotides long, which regulate gene expression post- transciptionally. miRNAs typically function by nega- tively regulating mRNA processing, stability, and trans- lation and thereby represent a novel layer in regulation of flow of genetic information and cellular functions. miRNAs are encoded in genomic DNA and are tran- scribed by RNA polymerase II into long primary tran- scripts called pri-miRNA. This pri-miRNA is sequen- tially processed in the cell nucleus and then in the cyto- plasm to generate mature active miRNA [17]. Recent evidence suggests that miRNA expression maybe impli- cated in several developmental, inflammatory, apoptotic, and cellular signal transduction pathways affecting mul- tiple disease pathogenesis, including cardiovascular dis- ease, cancer, metabolic diseases, lung development, and respiratory disease, etc. [18-25]. 3. Common Air Pollutants 3.1. Polycyclic Aromatic Hydrocarbons (PAH) Polycyclic aromatic hydrocarbons (PAHs) represent a complex class of environmental pollutants derived from the incomplete combustion of organic compounds. These relatively stable compounds are comprised of multiple, fused, benzene rings. The major sources of PAH include burning of biomass, wildfires, and vehicular emissions [26,27]. The primary sources of PAH production vary from country to country, with developing nations respon- sible for greater emissions than developed nations due in large part to disparities in production technology [27]. Exposure to PAH is most significant in urban regions marked by high levels of both industrial development as well as traffic [26,27]; significant global contributors include China, India, and Brazil [27]. Several studies have assessed occupational health risks in individuals exposed to disproportionate levels of PAHs, such as traffic controllers and industrial workers [28-31]. These studies show that PAH can be a potent carcinogen and has also been linked to adverse respiratory effects [26,27,32,33]. Additionally, PAH exposure has been as- sociated with the activation of DNA damage signaling, with multiple PAHs exerting more extensive damage than one polycyclic compound alone [34]. PAH exposure can begin in the womb and, through epigenetic modifications, can have long-lasting effects. Increased maternal exposure to PAH has been associated with increased DNA methylation of the asthma related gene acyl-CoA synthestase long-chain family member 3 (ACSL3) and subsequent increases in the prevalence of childhood asthma [8]. In another study, in vitro exposure was associated with hypermethylation of the asthma- Copyright © 2013 SciRes. JEP
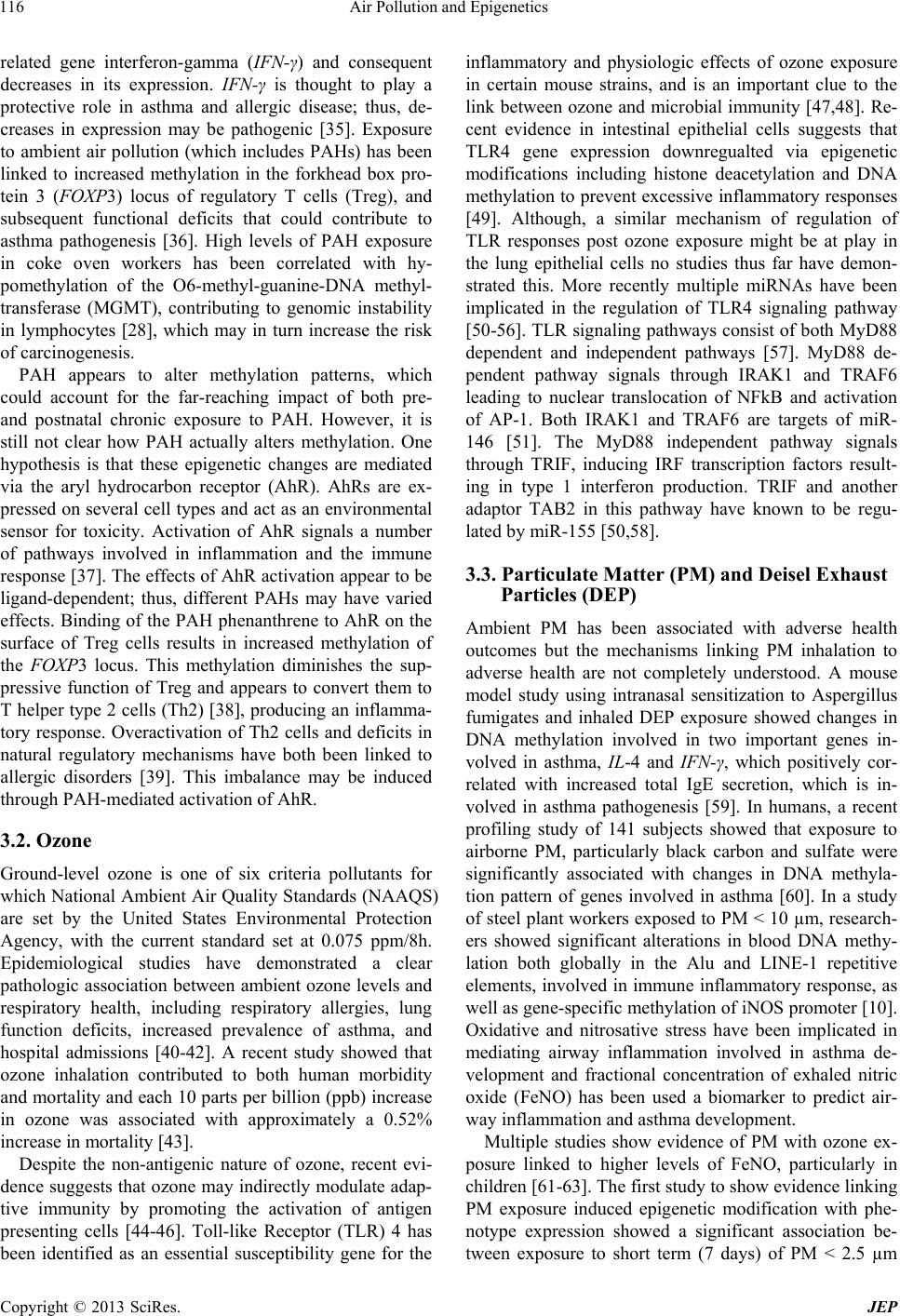 Air Pollution and Epigenetics 116 related gene interferon-gamma (IFN-γ) and consequent decreases in its expression. IFN-γ is thought to play a protective role in asthma and allergic disease; thus, de- creases in expression may be pathogenic [35]. Exposure to ambient air pollution (which includes PAHs) has been linked to increased methylation in the forkhead box pro- tein 3 (FOX P3) locus of regulatory T cells (Treg), and subsequent functional deficits that could contribute to asthma pathogenesis [36]. High levels of PAH exposure in coke oven workers has been correlated with hy- pomethylation of the O6-methyl-guanine-DNA methyl- transferase (MGMT), contributing to genomic instability in lymphocytes [28], which may in turn increase the risk of carcinogenesis. PAH appears to alter methylation patterns, which could account for the far-reaching impact of both pre- and postnatal chronic exposure to PAH. However, it is still not clear how PAH actually alters methylation. One hypothesis is that these epigenetic changes are mediated via the aryl hydrocarbon receptor (AhR). AhRs are ex- pressed on several cell types and act as an environmental sensor for toxicity. Activation of AhR signals a number of pathways involved in inflammation and the immune response [37]. The effects of AhR activation appear to be ligand-dependent; thus, different PAHs may have varied effects. Binding of the PAH phenanthrene to AhR on the surface of Treg cells results in increased methylation of the FOXP3 locus. This methylation diminishes the sup- pressive function of Treg and appears to convert them to T helper type 2 cells (Th2) [38], producing an inflamma- tory response. Overactivation of Th2 cells and deficits in natural regulatory mechanisms have both been linked to allergic disorders [39]. This imbalance may be induced through PAH-mediated activation of AhR. 3.2. Ozone Ground-level ozone is one of six criteria pollutants for which National Ambient Air Quality Standards (NAAQS) are set by the United States Environmental Protection Agency, with the current standard set at 0.075 ppm/8h. Epidemiological studies have demonstrated a clear pathologic association between ambient ozone levels and respiratory health, including respiratory allergies, lung function deficits, increased prevalence of asthma, and hospital admissions [40-42]. A recent study showed that ozone inhalation contributed to both human morbidity and mortality and each 10 parts per billion (ppb) increase in ozone was associated with approximately a 0.52% increase in mortality [43]. Despite the non-antigenic nature of ozone, recent evi- dence suggests that ozone may indirectly modulate adap- tive immunity by promoting the activation of antigen presenting cells [44-46]. Toll-like Receptor (TLR) 4 has been identified as an essential susceptibility gene for the inflammatory and physiologic effects of ozone exposure in certain mouse strains, and is an important clue to the link between ozone and microbial immunity [47,48]. Re- cent evidence in intestinal epithelial cells suggests that TLR4 gene expression downregualted via epigenetic modifications including histone deacetylation and DNA methylation to prevent excessive inflammatory responses [49]. Although, a similar mechanism of regulation of TLR responses post ozone exposure might be at play in the lung epithelial cells no studies thus far have demon- strated this. More recently multiple miRNAs have been implicated in the regulation of TLR4 signaling pathway [50-56]. TLR signaling pathways consist of both MyD88 dependent and independent pathways [57]. MyD88 de- pendent pathway signals through IRAK1 and TRAF6 leading to nuclear translocation of NFkB and activation of AP-1. Both IRAK1 and TRAF6 are targets of miR- 146 [51]. The MyD88 independent pathway signals through TRIF, inducing IRF transcription factors result- ing in type 1 interferon production. TRIF and another adaptor TAB2 in this pathway have known to be regu- lated by miR-155 [50,58]. 3.3. Particulate Matter (PM) and Deisel Exhaust Particles (DEP) Ambient PM has been associated with adverse health outcomes but the mechanisms linking PM inhalation to adverse health are not completely understood. A mouse model study using intranasal sensitization to Aspergillus fumigates and inhaled DEP exposure showed changes in DNA methylation involved in two important genes in- volved in asthma, IL-4 and IFN-γ, which positively cor- related with increased total IgE secretion, which is in- volved in asthma pathogenesis [59]. In humans, a recent profiling study of 141 subjects showed that exposure to airborne PM, particularly black carbon and sulfate were significantly associated with changes in DNA methyla- tion pattern of genes involved in asthma [60]. In a study of steel plant workers exposed to PM < 10 µm, research- ers showed significant alterations in blood DNA methy- lation both globally in the Alu and LINE-1 repetitive elements, involved in immune inflammatory response, as well as gene-specific methylation of iNOS promoter [10]. Oxidative and nitrosative stress have been implicated in mediating airway inflammation involved in asthma de- velopment and fractional concentration of exhaled nitric oxide (FeNO) has been used a biomarker to predict air- way inflammation and asthma development. Multiple studies show evidence of PM with ozone ex- posure linked to higher levels of FeNO, particularly in children [61-63]. The first study to show evidence linking PM exposure induced epigenetic modification with phe- notype expression showed a significant association be- tween exposure to short term (7 days) of PM < 2.5 µm Copyright © 2013 SciRes. JEP
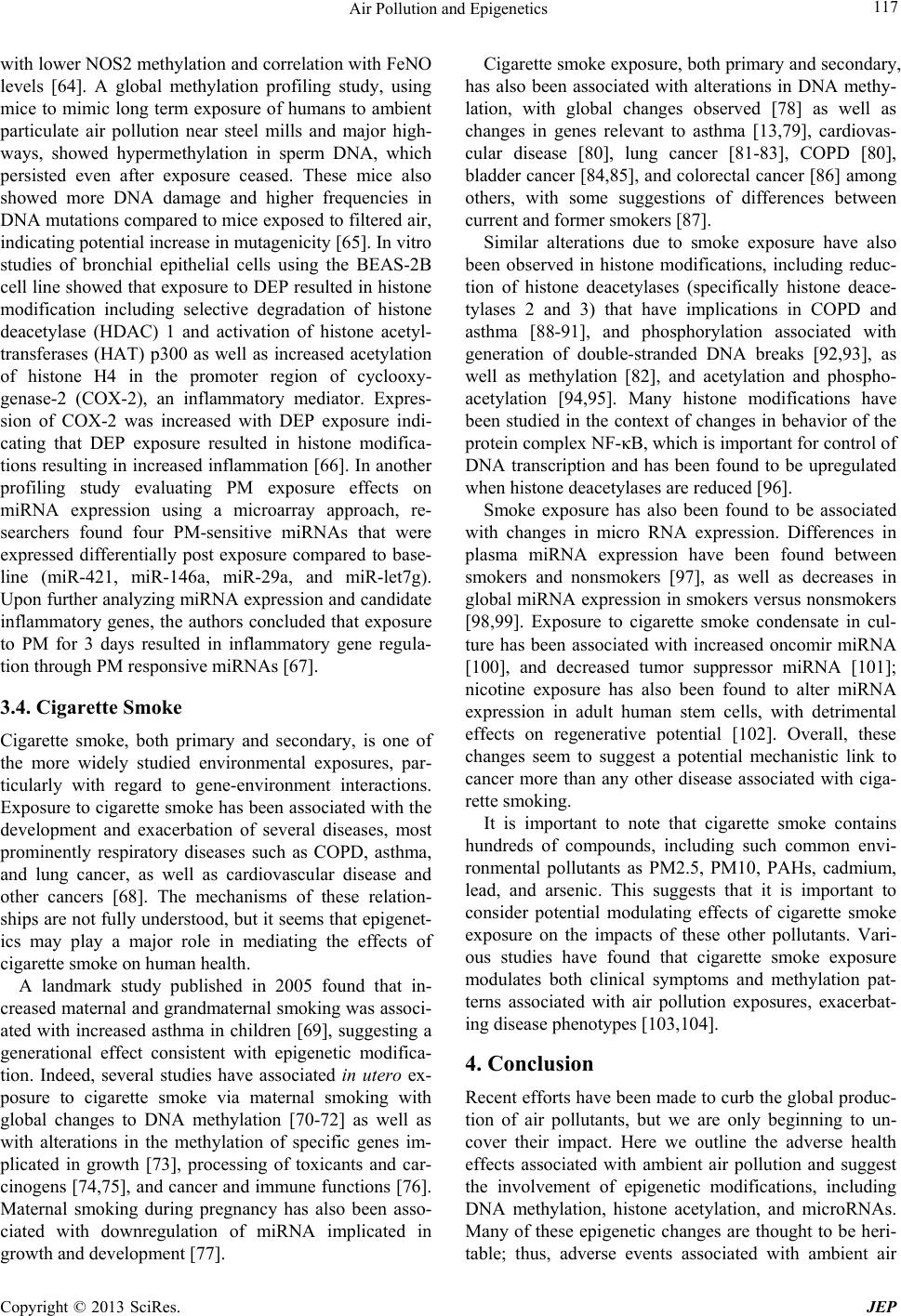 Air Pollution and Epigenetics 117 with lower NOS2 methylation and correlation with FeNO levels [64]. A global methylation profiling study, using mice to mimic long term exposure of humans to ambient particulate air pollution near steel mills and major high- ways, showed hypermethylation in sperm DNA, which persisted even after exposure ceased. These mice also showed more DNA damage and higher frequencies in DNA mutations compared to mice exposed to filtered air, indicating potential increase in mutagenicity [65]. In vitro studies of bronchial epithelial cells using the BEAS-2B cell line showed that exposure to DEP resulted in histone modification including selective degradation of histone deacetylase (HDAC) 1 and activation of histone acetyl- transferases (HAT) p300 as well as increased acetylation of histone H4 in the promoter region of cyclooxy- genase-2 (COX-2), an inflammatory mediator. Expres- sion of COX-2 was increased with DEP exposure indi- cating that DEP exposure resulted in histone modifica- tions resulting in increased inflammation [66]. In another profiling study evaluating PM exposure effects on miRNA expression using a microarray approach, re- searchers found four PM-sensitive miRNAs that were expressed differentially post exposure compared to base- line (miR-421, miR-146a, miR-29a, and miR-let7g). Upon further analyzing miRNA expression and candidate inflammatory genes, the authors concluded that exposure to PM for 3 days resulted in inflammatory gene regula- tion through PM responsive miRNAs [67]. 3.4. Cigarette Smoke Cigarette smoke, both primary and secondary, is one of the more widely studied environmental exposures, par- ticularly with regard to gene-environment interactions. Exposure to cigarette smoke has been associated with the development and exacerbation of several diseases, most prominently respiratory diseases such as COPD, asthma, and lung cancer, as well as cardiovascular disease and other cancers [68]. The mechanisms of these relation- ships are not fully understood, but it seems that epigenet- ics may play a major role in mediating the effects of cigarette smoke on human health. A landmark study published in 2005 found that in- creased maternal and grandmaternal smoking was associ- ated with increased asthma in children [69], suggesting a generational effect consistent with epigenetic modifica- tion. Indeed, several studies have associated in utero ex- posure to cigarette smoke via maternal smoking with global changes to DNA methylation [70-72] as well as with alterations in the methylation of specific genes im- plicated in growth [73], processing of toxicants and car- cinogens [74,75], and cancer and immune functions [76]. Maternal smoking during pregnancy has also been asso- ciated with downregulation of miRNA implicated in growth and development [77]. Cigarette smoke exposure, both primary and secondary, has also been associated with alterations in DNA methy- lation, with global changes observed [78] as well as changes in genes relevant to asthma [13,79], cardiovas- cular disease [80], lung cancer [81-83], COPD [80], bladder cancer [84,85], and colorectal cancer [86] among others, with some suggestions of differences between current and former smokers [87]. Similar alterations due to smoke exposure have also been observed in histone modifications, including reduc- tion of histone deacetylases (specifically histone deace- tylases 2 and 3) that have implications in COPD and asthma [88-91], and phosphorylation associated with generation of double-stranded DNA breaks [92,93], as well as methylation [82], and acetylation and phospho- acetylation [94,95]. Many histone modifications have been studied in the context of changes in behavior of the protein complex NF-κB, which is important for control of DNA transcription and has been found to be upregulated when histone deacetylases are reduced [96]. Smoke exposure has also been found to be associated with changes in micro RNA expression. Differences in plasma miRNA expression have been found between smokers and nonsmokers [97], as well as decreases in global miRNA expression in smokers versus nonsmokers [98,99]. Exposure to cigarette smoke condensate in cul- ture has been associated with increased oncomir miRNA [100], and decreased tumor suppressor miRNA [101]; nicotine exposure has also been found to alter miRNA expression in adult human stem cells, with detrimental effects on regenerative potential [102]. Overall, these changes seem to suggest a potential mechanistic link to cancer more than any other disease associated with ciga- rette smoking. It is important to note that cigarette smoke contains hundreds of compounds, including such common envi- ronmental pollutants as PM2.5, PM10, PAHs, cadmium, lead, and arsenic. This suggests that it is important to consider potential modulating effects of cigarette smoke exposure on the impacts of these other pollutants. Vari- ous studies have found that cigarette smoke exposure modulates both clinical symptoms and methylation pat- terns associated with air pollution exposures, exacerbat- ing disease phenotypes [103,104]. 4. Conclusion Recent efforts have been made to curb the global produc- tion of air pollutants, but we are only beginning to un- cover their impact. Here we outline the adverse health effects associated with ambient air pollution and suggest the involvement of epigenetic modifications, including DNA methylation, histone acetylation, and microRNAs. Many of these epigenetic changes are thought to be heri- table; thus, adverse events associated with ambient air Copyright © 2013 SciRes. JEP
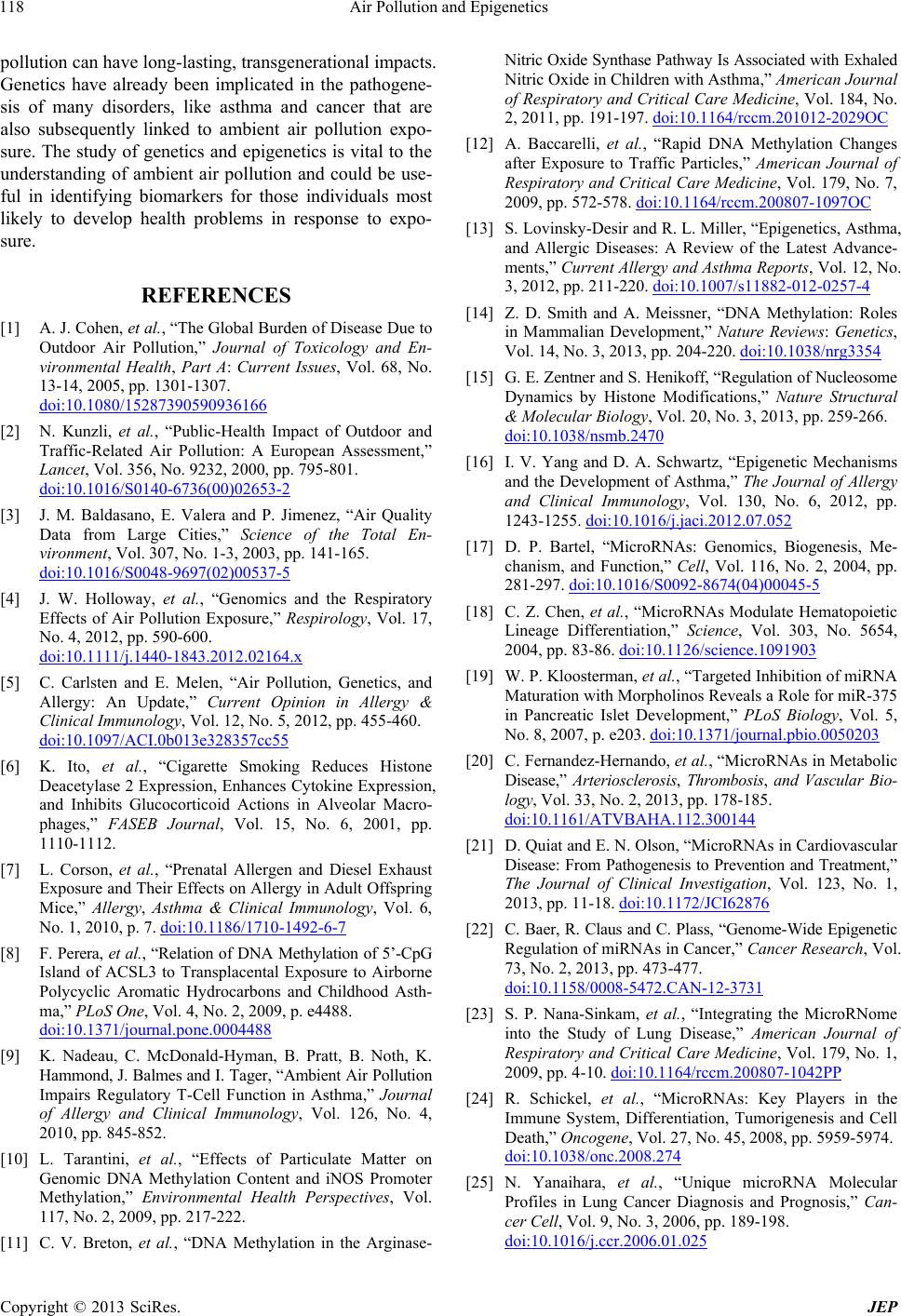 Air Pollution and Epigenetics 118 pollution can have long-lasting, transgenerational impacts. Genetics have already been implicated in the pathogene- sis of many disorders, like asthma and cancer that are also subsequently linked to ambient air pollution expo- sure. The study of genetics and epigenetics is vital to the understanding of ambient air pollution and could be use- ful in identifying biomarkers for those individuals most likely to develop health problems in response to expo- sure. REFERENCES [1] A. J. Cohen, et al., “The Global Burden of Disease Due to Outdoor Air Pollution,” Journal of Toxicology and En- vironmental Health, Part A: Current Issues, Vol. 68, No. 13-14, 2005, pp. 1301-1307. doi:10.1080/15287390590936166 [2] N. Kunzli, et al., “Public-Health Impact of Outdoor and Traffic-Related Air Pollution: A European Assessment,” Lancet, Vol. 356, No. 9232, 2000, pp. 795-801. doi:10.1016/S0140-6736(00)02653-2 [3] J. M. Baldasano, E. Valera and P. Jimenez, “Air Quality Data from Large Cities,” Science of the Total En- vironment, Vol. 307, No. 1-3, 2003, pp. 141-165. doi:10.1016/S0048-9697(02)00537-5 [4] J. W. Holloway, et al., “Genomics and the Respiratory Effects of Air Pollution Exposure,” Respirology, Vol. 17, No. 4, 2012, pp. 590-600. doi:10.1111/j.1440-1843.2012.02164.x [5] C. Carlsten and E. Melen, “Air Pollution, Genetics, and Allergy: An Update,” Current Opinion in Allergy & Clinical Immunology, Vol. 12, No. 5, 2012, pp. 455-460. doi:10.1097/ACI.0b013e328357cc55 [6] K. Ito, et al., “Cigarette Smoking Reduces Histone Deacetylase 2 Expression, Enhances Cytokine Expression, and Inhibits Glucocorticoid Actions in Alveolar Macro- phages,” FASEB Journal, Vol. 15, No. 6, 2001, pp. 1110-1112. [7] L. Corson, et al., “Prenatal Allergen and Diesel Exhaust Exposure and Their Effects on Allergy in Adult Offspring Mice,” Allergy, Asthma & Clinical Immunology, Vol. 6, No. 1, 2010, p. 7. doi:10.1186/1710-1492-6-7 [8] F. Perera, et al., “Relation of DNA Methylation of 5’-CpG Island of ACSL3 to Transplacental Exposure to Airborne Polycyclic Aromatic Hydrocarbons and Childhood Asth- ma,” PLoS One, Vol. 4, No. 2, 2009, p. e4488. doi:10.1371/journal.pone.0004488 [9] K. Nadeau, C. McDonald-Hyman, B. Pratt, B. Noth, K. Hammond, J. Balmes and I. Tager, “Ambient Air Pollution Impairs Regulatory T-Cell Function in Asthma,” Journal of Allergy and Clinical Immunology, Vol. 126, No. 4, 2010, pp. 845-852. [10] L. Tarantini, et al., “Effects of Particulate Matter on Genomic DNA Methylation Content and iNOS Promoter Methylation,” Environmental Health Perspectives, Vol. 117, No. 2, 2009, pp. 217-222. [11] C. V. Breton, et al., “DNA Methylation in the Arginase- Nitric Oxide Synthase Pathway Is Associated with Exhaled Nitric Oxide in Children with Asthma,” American Journal of Respiratory and Critical Care Medicine, Vol. 184, No. 2, 2011, pp. 191-197. doi:10.1164/rccm.201012-2029OC [12] A. Baccarelli, et al., “Rapid DNA Methylation Changes after Exposure to Traffic Particles,” American Journal of Respiratory and Critical Care Medicine, Vol. 179, No. 7, 2009, pp. 572-578. doi:10.1164/rccm.200807-1097OC [13] S. Lovinsky-Desir and R. L. Miller, “Epigenetics, Asthma, and Allergic Diseases: A Review of the Latest Advance- ments,” Current Allergy and Asthma Reports, Vol. 12, No. 3, 2012, pp. 211-220. doi:10.1007/s11882-012-0257-4 [14] Z. D. Smith and A. Meissner, “DNA Methylation: Roles in Mammalian Development,” Nature Reviews: Genetics, Vol. 14, No. 3, 2013, pp. 204-220. doi:10.1038/nrg3354 [15] G. E. Zentner and S. Henikoff, “Regulation of Nucleosome Dynamics by Histone Modifications,” Nature Structural & Molecular Biology, Vol. 20, No. 3, 2013, pp. 259-266. doi:10.1038/nsmb.2470 [16] I. V. Yang and D. A. Schwartz, “Epigenetic Mechanisms and the Development of Asthma,” The Journal of Allergy and Clinical Immunology, Vol. 130, No. 6, 2012, pp. 1243-1255. doi:10.1016/j.jaci.2012.07.052 [17] D. P. Bartel, “MicroRNAs: Genomics, Biogenesis, Me- chanism, and Function,” Cell, Vol. 116, No. 2, 2004, pp. 281-297. doi:10.1016/S0092-8674(04)00045-5 [18] C. Z. Chen, et al., “MicroRNAs Modulate Hematopoietic Lineage Differentiation,” Science, Vol. 303, No. 5654, 2004, pp. 83-86. doi:10.1126/science.1091903 [19] W. P. Kloosterman, et al., “Targeted Inhibition of miRNA Maturation with Morpholinos Reveals a Role for miR-375 in Pancreatic Islet Development,” PLoS Biology, Vol. 5, No. 8, 2007, p. e203. doi:10.1371/journal.pbio.0050203 [20] C. Fernandez-Hernando, et al., “MicroRNAs in Metabolic Disease,” Arteriosclerosis, Thrombosis, and Vascular Bio- logy, Vol. 33, No. 2, 2013, pp. 178-185. doi:10.1161/ATVBAHA.112.300144 [21] D. Quiat and E. N. Olson, “MicroRNAs in Cardiovascular Disease: From Pathogenesis to Prevention and Treatment,” The Journal of Clinical Investigation, Vol. 123, No. 1, 2013, pp. 11-18. doi:10.1172/JCI62876 [22] C. Baer, R. Claus and C. Plass, “Genome-Wide Epigenetic Regulation of miRNAs in Cancer,” Cancer Research, Vol. 73, No. 2, 2013, pp. 473-477. doi:10.1158/0008-5472.CAN-12-3731 [23] S. P. Nana-Sinkam, et al., “Integrating the MicroRNome into the Study of Lung Disease,” American Journal of Respiratory and Critical Care Medicine, Vol. 179, No. 1, 2009, pp. 4-10. doi:10.1164/rccm.200807-1042PP [24] R. Schickel, et al., “MicroRNAs: Key Players in the Immune System, Differentiation, Tumorigenesis and Cell Death,” Oncogene, Vol. 27, No. 45, 2008, pp. 5959-5974. doi:10.1038/onc.2008.274 [25] N. Yanaihara, et al., “Unique microRNA Molecular Profiles in Lung Cancer Diagnosis and Prognosis,” Can- cer Cell, Vol. 9, No. 3, 2006, pp. 189-198. doi:10.1016/j.ccr.2006.01.025 Copyright © 2013 SciRes. JEP
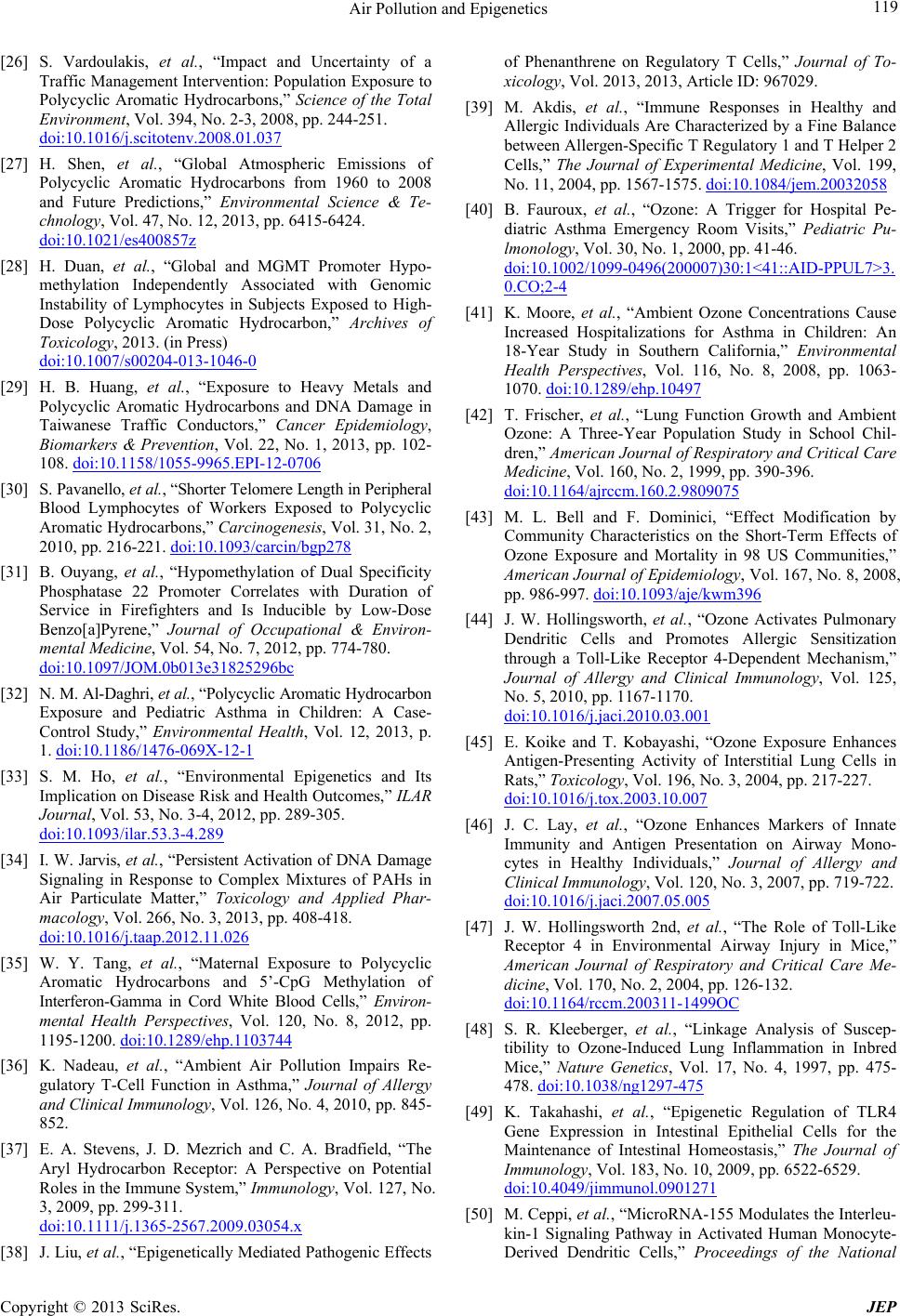 Air Pollution and Epigenetics 119 [26] S. Vardoulakis, et al., “Impact and Uncertainty of a Traffic Management Intervention: Population Exposure to Polycyclic Aromatic Hydrocarbons,” Science of the Total Environment, Vol. 394, No. 2-3, 2008, pp. 244-251. doi:10.1016/j.scitotenv.2008.01.037 [27] H. Shen, et al., “Global Atmospheric Emissions of Polycyclic Aromatic Hydrocarbons from 1960 to 2008 and Future Predictions,” Environmental Science & Te- chnology, Vol. 47, No. 12, 2013, pp. 6415-6424. doi:10.1021/es400857z [28] H. Duan, et al., “Global and MGMT Promoter Hypo- methylation Independently Associated with Genomic Instability of Lymphocytes in Subjects Exposed to High- Dose Polycyclic Aromatic Hydrocarbon,” Archives of Toxicology, 2013. (in Press) doi:10.1007/s00204-013-1046-0 [29] H. B. Huang, et al., “Exposure to Heavy Metals and Polycyclic Aromatic Hydrocarbons and DNA Damage in Taiwanese Traffic Conductors,” Cancer Epidemiology, Biomarkers & Prevention, Vol. 22, No. 1, 2013, pp. 102- 108. doi:10.1158/1055-9965.EPI-12-0706 [30] S. Pavanello, et al., “Shorter Telomere Length in Peripheral Blood Lymphocytes of Workers Exposed to Polycyclic Aromatic Hydrocarbons,” Carcinogenesis, Vol. 31, No. 2, 2010, pp. 216-221. doi:10.1093/carcin/bgp278 [31] B. Ouyang, et al., “Hypomethylation of Dual Specificity Phosphatase 22 Promoter Correlates with Duration of Service in Firefighters and Is Inducible by Low-Dose Benzo[a]Pyrene,” Journal of Occupational & Environ- mental Medicine, Vol. 54, No. 7, 2012, pp. 774-780. doi:10.1097/JOM.0b013e31825296bc [32] N. M. Al-Daghri, et al., “Polycyclic Aromatic Hydrocarbon Exposure and Pediatric Asthma in Children: A Case- Control Study,” Environmental Health, Vol. 12, 2013, p. 1. doi:10.1186/1476-069X-12-1 [33] S. M. Ho, et al., “Environmental Epigenetics and Its Implication on Disease Risk and Health Outcomes,” ILAR Journal, Vol. 53, No. 3-4, 2012, pp. 289-305. doi:10.1093/ilar.53.3-4.289 [34] I. W. Jarvis, et al., “Persistent Activation of DNA Damage Signaling in Response to Complex Mixtures of PAHs in Air Particulate Matter,” Toxicology and Applied Phar- macology, Vol. 266, No. 3, 2013, pp. 408-418. doi:10.1016/j.taap.2012.11.026 [35] W. Y. Tang, et al., “Maternal Exposure to Polycyclic Aromatic Hydrocarbons and 5’-CpG Methylation of Interferon-Gamma in Cord White Blood Cells,” Environ- mental Health Perspectives, Vol. 120, No. 8, 2012, pp. 1195-1200. doi:10.1289/ehp.1103744 [36] K. Nadeau, et al., “Ambient Air Pollution Impairs Re- gulatory T-Cell Function in Asthma,” Journal of Allergy and Clinical Immunology, Vol. 126, No. 4, 2010, pp. 845- 852. [37] E. A. Stevens, J. D. Mezrich and C. A. Bradfield, “The Aryl Hydrocarbon Receptor: A Perspective on Potential Roles in the Immune System,” Immunology, Vol. 127, No. 3, 2009, pp. 299-311. doi:10.1111/j.1365-2567.2009.03054.x [38] J. Liu, et al., “Epigenetically Mediated Pathogenic Effects of Phenanthrene on Regulatory T Cells,” Journal of To- xicology, Vol. 2013, 2013, Article ID: 967029. [39] M. Akdis, et al., “Immune Responses in Healthy and Allergic Individuals Are Characterized by a Fine Balance between Allergen-Specific T Regulatory 1 and T Helper 2 Cells,” The Journal of Experimental Medicine, Vol. 199, No. 11, 2004, pp. 1567-1575. doi:10.1084/jem.20032058 [40] B. Fauroux, et al., “Ozone: A Trigger for Hospital Pe- diatric Asthma Emergency Room Visits,” Pediatric Pu- lmonology, Vol. 30, No. 1, 2000, pp. 41-46. doi:10.1002/1099-0496(200007)30:1<41::AID-PPUL7>3. 0.CO;2-4 [41] K. Moore, et al., “Ambient Ozone Concentrations Cause Increased Hospitalizations for Asthma in Children: An 18-Year Study in Southern California,” Environmental Health Perspectives, Vol. 116, No. 8, 2008, pp. 1063- 1070. doi:10.1289/ehp.10497 [42] T. Frischer, et al., “Lung Function Growth and Ambient Ozone: A Three-Year Population Study in School Chil- dren,” American Journal of Respiratory and Critical Care Medicine, Vol. 160, No. 2, 1999, pp. 390-396. doi:10.1164/ajrccm.160.2.9809075 [43] M. L. Bell and F. Dominici, “Effect Modification by Community Characteristics on the Short-Term Effects of Ozone Exposure and Mortality in 98 US Communities,” American Journal of Epidemiology, Vol. 167, No. 8, 2008, pp. 986-997. doi:10.1093/aje/kwm396 [44] J. W. Hollingsworth, et al., “Ozone Activates Pulmonary Dendritic Cells and Promotes Allergic Sensitization through a Toll-Like Receptor 4-Dependent Mechanism,” Journal of Allergy and Clinical Immunology, Vol. 125, No. 5, 2010, pp. 1167-1170. doi:10.1016/j.jaci.2010.03.001 [45] E. Koike and T. Kobayashi, “Ozone Exposure Enhances Antigen-Presenting Activity of Interstitial Lung Cells in Rats,” Toxicology, Vol. 196, No. 3, 2004, pp. 217-227. doi:10.1016/j.tox.2003.10.007 [46] J. C. Lay, et al., “Ozone Enhances Markers of Innate Immunity and Antigen Presentation on Airway Mono- cytes in Healthy Individuals,” Journal of Allergy and Clinical Immunology, Vol. 120, No. 3, 2007, pp. 719-722. doi:10.1016/j.jaci.2007.05.005 [47] J. W. Hollingsworth 2nd, et al., “The Role of Toll-Like Receptor 4 in Environmental Airway Injury in Mice,” American Journal of Respiratory and Critical Care Me- dicine, Vol. 170, No. 2, 2004, pp. 126-132. doi:10.1164/rccm.200311-1499OC [48] S. R. Kleeberger, et al., “Linkage Analysis of Suscep- tibility to Ozone-Induced Lung Inflammation in Inbred Mice,” Nature Genetics, Vol. 17, No. 4, 1997, pp. 475- 478. doi:10.1038/ng1297-475 [49] K. Takahashi, et al., “Epigenetic Regulation of TLR4 Gene Expression in Intestinal Epithelial Cells for the Maintenance of Intestinal Homeostasis,” The Journal of Immunology, Vol. 183, No. 10, 2009, pp. 6522-6529. doi:10.4049/jimmunol.0901271 [50] M. Ceppi, et al., “MicroRNA-155 Modulates the Interleu- kin-1 Signaling Pathway in Activated Human Monocyte- Derived Dendritic Cells,” Proceedings of the National Copyright © 2013 SciRes. JEP
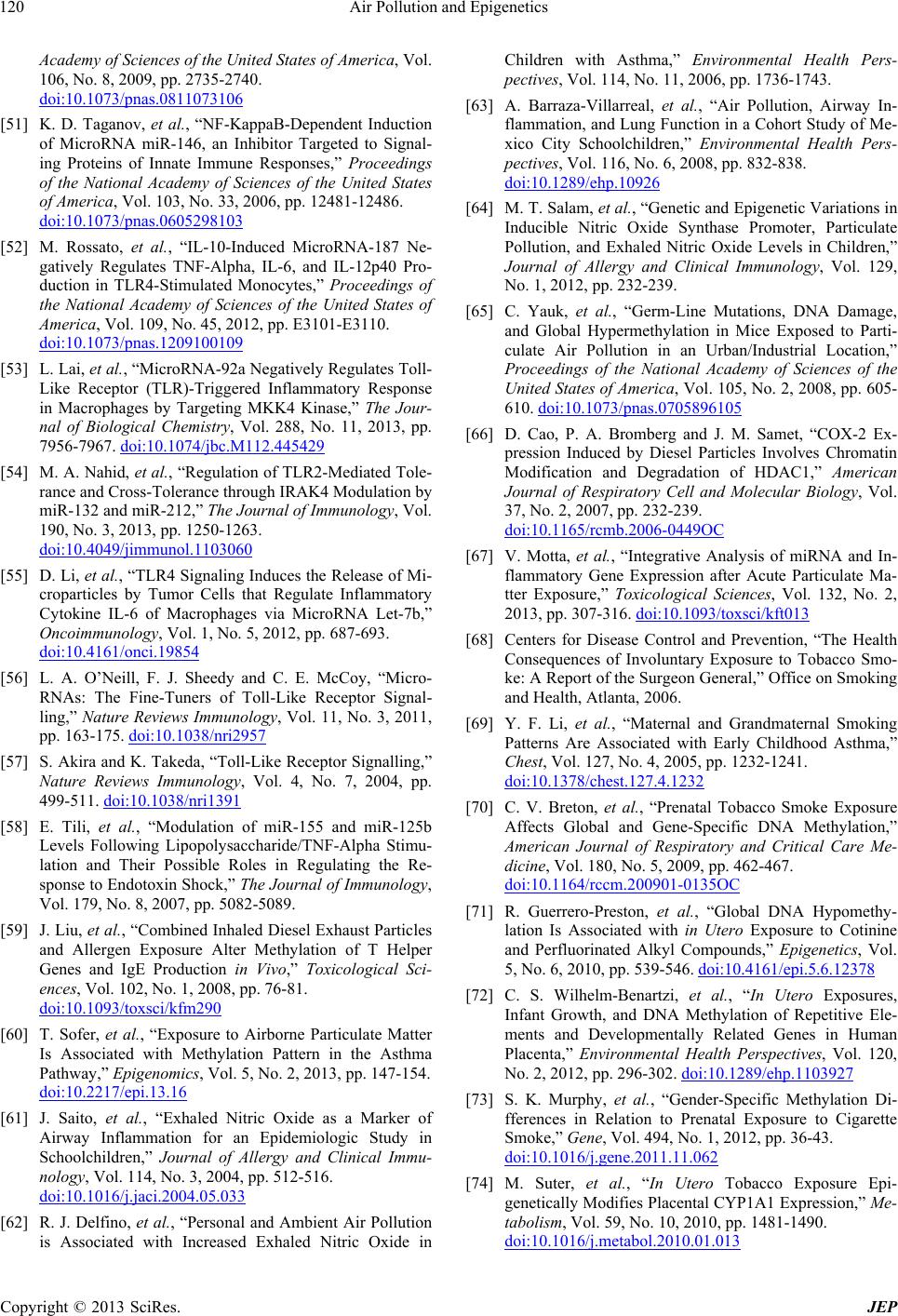 Air Pollution and Epigenetics 120 Academy of Sciences of the United States of America, Vol. 106, No. 8, 2009, pp. 2735-2740. doi:10.1073/pnas.0811073106 [51] K. D. Taganov, et al., “NF-KappaB-Dependent Induction of MicroRNA miR-146, an Inhibitor Targeted to Signal- ing Proteins of Innate Immune Responses,” Proceedings of the National Academy of Sciences of the United States of America, Vol. 103, No. 33, 2006, pp. 12481-12486. doi:10.1073/pnas.0605298103 [52] M. Rossato, et al., “IL-10-Induced MicroRNA-187 Ne- gatively Regulates TNF-Alpha, IL-6, and IL-12p40 Pro- duction in TLR4-Stimulated Monocytes,” Proceedings of the National Academy of Sciences of the United States of America, Vol. 109, No. 45, 2012, pp. E3101-E3110. doi:10.1073/pnas.1209100109 [53] L. Lai, et al., “MicroRNA-92a Negatively Regulates Toll- Like Receptor (TLR)-Triggered Inflammatory Response in Macrophages by Targeting MKK4 Kinase,” The Jour- nal of Biological Chemistry, Vol. 288, No. 11, 2013, pp. 7956-7967. doi:10.1074/jbc.M112.445429 [54] M. A. Nahid, et al., “Regulation of TLR2-Mediated Tole- rance and Cross-Tolerance through IRAK4 Modulation by miR-132 and miR-212,” The Journal of Immunology, Vol. 190, No. 3, 2013, pp. 1250-1263. doi:10.4049/jimmunol.1103060 [55] D. Li, et al., “TLR4 Signaling Induces the Release of Mi- croparticles by Tumor Cells that Regulate Inflammatory Cytokine IL-6 of Macrophages via MicroRNA Let-7b,” Oncoimmunology, Vol. 1, No. 5, 2012, pp. 687-693. doi:10.4161/onci.19854 [56] L. A. O’Neill, F. J. Sheedy and C. E. McCoy, “Micro- RNAs: The Fine-Tuners of Toll-Like Receptor Signal- ling,” Nature Reviews Immunology, Vol. 11, No. 3, 2011, pp. 163-175. doi:10.1038/nri2957 [57] S. Akira and K. Takeda, “Toll-Like Receptor Signalling,” Nature Reviews Immunology, Vol. 4, No. 7, 2004, pp. 499-511. doi:10.1038/nri1391 [58] E. Tili, et al., “Modulation of miR-155 and miR-125b Levels Following Lipopolysaccharide/TNF-Alpha Stimu- lation and Their Possible Roles in Regulating the Re- sponse to Endotoxin Shock,” The Journal of Immunology, Vol. 179, No. 8, 2007, pp. 5082-5089. [59] J. Liu, et al., “Combined Inhaled Diesel Exhaust Particles and Allergen Exposure Alter Methylation of T Helper Genes and IgE Production in Vivo,” Toxicological Sci- ences, Vol. 102, No. 1, 2008, pp. 76-81. doi:10.1093/toxsci/kfm290 [60] T. Sofer, et al., “Exposure to Airborne Particulate Matter Is Associated with Methylation Pattern in the Asthma Pathway,” Epigenomics, Vol. 5, No. 2, 2013, pp. 147-154. doi:10.2217/epi.13.16 [61] J. Saito, et al., “Exhaled Nitric Oxide as a Marker of Airway Inflammation for an Epidemiologic Study in Schoolchildren,” Journal of Allergy and Clinical Immu- nology, Vol. 114, No. 3, 2004, pp. 512-516. doi:10.1016/j.jaci.2004.05.033 [62] R. J. Delfino, et al., “Personal and Ambient Air Pollution is Associated with Increased Exhaled Nitric Oxide in Children with Asthma,” Environmental Health Pers- pectives, Vol. 114, No. 11, 2006, pp. 1736-1743. [63] A. Barraza-Villarreal, et al., “Air Pollution, Airway In- flammation, and Lung Function in a Cohort Study of Me- xico City Schoolchildren,” Environmental Health Pers- pectives, Vol. 116, No. 6, 2008, pp. 832-838. doi:10.1289/ehp.10926 [64] M. T. Salam, et al., “Genetic and Epigenetic Variations in Inducible Nitric Oxide Synthase Promoter, Particulate Pollution, and Exhaled Nitric Oxide Levels in Children,” Journal of Allergy and Clinical Immunology, Vol. 129, No. 1, 2012, pp. 232-239. [65] C. Yauk, et al., “Germ-Line Mutations, DNA Damage, and Global Hypermethylation in Mice Exposed to Parti- culate Air Pollution in an Urban/Industrial Location,” Proceedings of the National Academy of Sciences of the United States of America, Vol. 105, No. 2, 2008, pp. 605- 610. doi:10.1073/pnas.0705896105 [66] D. Cao, P. A. Bromberg and J. M. Samet, “COX-2 Ex- pression Induced by Diesel Particles Involves Chromatin Modification and Degradation of HDAC1,” American Journal of Respiratory Cell and Molecular Biology, Vol. 37, No. 2, 2007, pp. 232-239. doi:10.1165/rcmb.2006-0449OC [67] V. Motta, et al., “Integrative Analysis of miRNA and In- flammatory Gene Expression after Acute Particulate Ma- tter Exposure,” Toxicological Sciences, Vol. 132, No. 2, 2013, pp. 307-316. doi:10.1093/toxsci/kft013 [68] Centers for Disease Control and Prevention, “The Health Consequences of Involuntary Exposure to Tobacco Smo- ke: A Report of the Surgeon General,” Office on Smoking and Health, Atlanta, 2006. [69] Y. F. Li, et al., “Maternal and Grandmaternal Smoking Patterns Are Associated with Early Childhood Asthma,” Chest, Vol. 127, No. 4, 2005, pp. 1232-1241. doi:10.1378/chest.127.4.1232 [70] C. V. Breton, et al., “Prenatal Tobacco Smoke Exposure Affects Global and Gene-Specific DNA Methylation,” American Journal of Respiratory and Critical Care Me- dicine, Vol. 180, No. 5, 2009, pp. 462-467. doi:10.1164/rccm.200901-0135OC [71] R. Guerrero-Preston, et al., “Global DNA Hypomethy- lation Is Associated with in Utero Exposure to Cotinine and Perfluorinated Alkyl Compounds,” Epigenetics, Vol. 5, No. 6, 2010, pp. 539-546. doi:10.4161/epi.5.6.12378 [72] C. S. Wilhelm-Benartzi, et al., “In Utero Exposures, Infant Growth, and DNA Methylation of Repetitive Ele- ments and Developmentally Related Genes in Human Placenta,” Environmental Health Perspectives, Vol. 120, No. 2, 2012, pp. 296-302. doi:10.1289/ehp.1103927 [73] S. K. Murphy, et al., “Gender-Specific Methylation Di- fferences in Relation to Prenatal Exposure to Cigarette Smoke,” Gene, Vol. 494, No. 1, 2012, pp. 36-43. doi:10.1016/j.gene.2011.11.062 [74] M. Suter, et al., “In Utero Tobacco Exposure Epi- genetically Modifies Placental CYP1A1 Expression,” Me- tabolism, Vol. 59, No. 10, 2010, pp. 1481-1490. doi:10.1016/j.metabol.2010.01.013 Copyright © 2013 SciRes. JEP
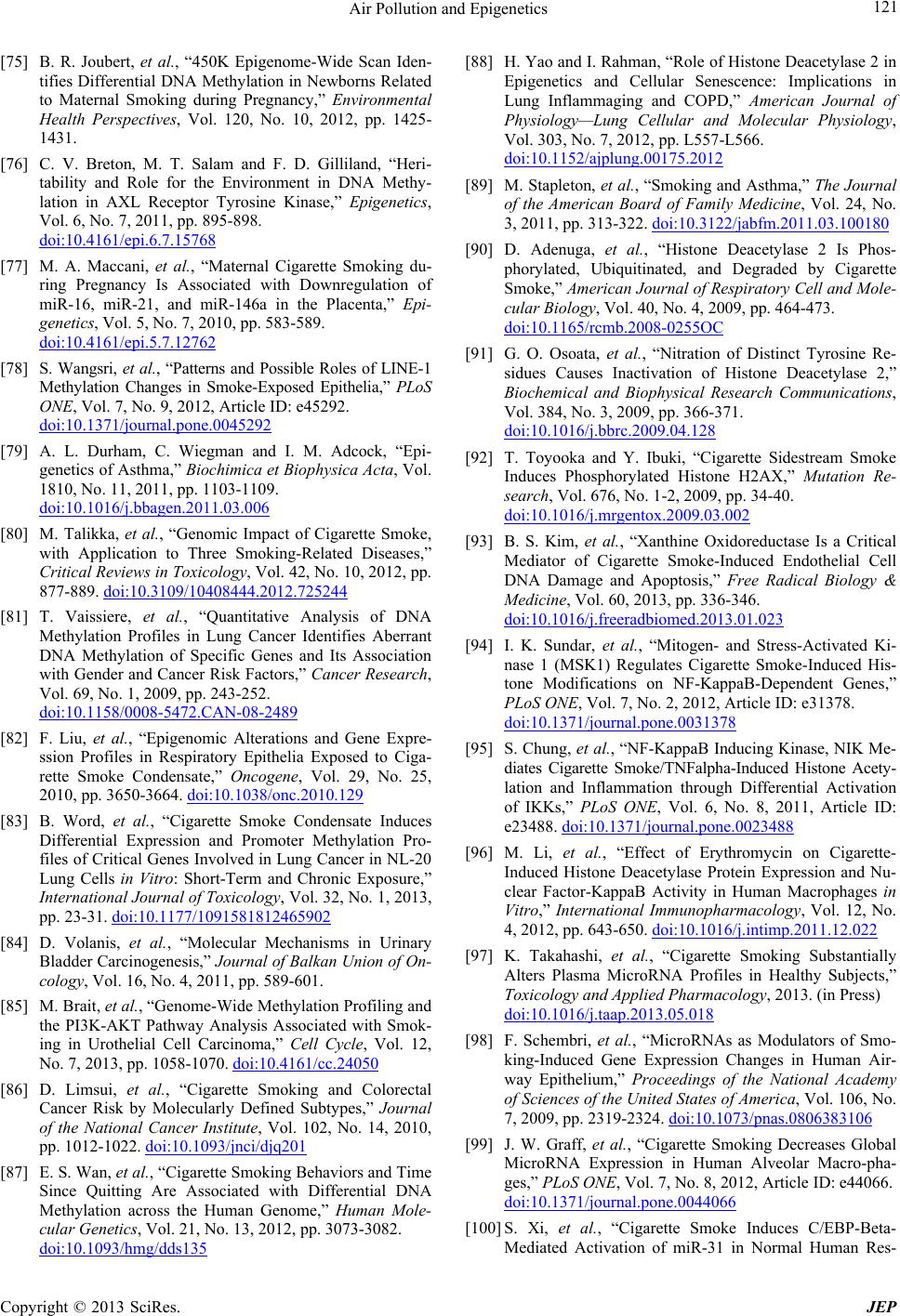 Air Pollution and Epigenetics 121 [75] B. R. Joubert, et al., “450K Epigenome-Wide Scan Iden- tifies Differential DNA Methylation in Newborns Related to Maternal Smoking during Pregnancy,” Environmental Health Perspectives, Vol. 120, No. 10, 2012, pp. 1425- 1431. [76] C. V. Breton, M. T. Salam and F. D. Gilliland, “Heri- tability and Role for the Environment in DNA Methy- lation in AXL Receptor Tyrosine Kinase,” Epigenetics, Vol. 6, No. 7, 2011, pp. 895-898. doi:10.4161/epi.6.7.15768 [77] M. A. Maccani, et al., “Maternal Cigarette Smoking du- ring Pregnancy Is Associated with Downregulation of miR-16, miR-21, and miR-146a in the Placenta,” Epi- genetics, Vol. 5, No. 7, 2010, pp. 583-589. doi:10.4161/epi.5.7.12762 [78] S. Wangsri, et al., “Patterns and Possible Roles of LINE-1 Methylation Changes in Smoke-Exposed Epithelia,” PLoS ONE, Vol. 7, No. 9, 2012, Article ID: e45292. doi:10.1371/journal.pone.0045292 [79] A. L. Durham, C. Wiegman and I. M. Adcock, “Epi- genetics of Asthma,” Biochimica et Biophysica Acta, Vol. 1810, No. 11, 2011, pp. 1103-1109. doi:10.1016/j.bbagen.2011.03.006 [80] M. Talikka, et al., “Genomic Impact of Cigarette Smoke, with Application to Three Smoking-Related Diseases,” Critical Reviews in Toxicology, Vol. 42, No. 10, 2012, pp. 877-889. doi:10.3109/10408444.2012.725244 [81] T. Vaissiere, et al., “Quantitative Analysis of DNA Methylation Profiles in Lung Cancer Identifies Aberrant DNA Methylation of Specific Genes and Its Association with Gender and Cancer Risk Factors,” Cancer Research, Vol. 69, No. 1, 2009, pp. 243-252. doi:10.1158/0008-5472.CAN-08-2489 [82] F. Liu, et al., “Epigenomic Alterations and Gene Expre- ssion Profiles in Respiratory Epithelia Exposed to Ciga- rette Smoke Condensate,” Oncogene, Vol. 29, No. 25, 2010, pp. 3650-3664. doi:10.1038/onc.2010.129 [83] B. Word, et al., “Cigarette Smoke Condensate Induces Differential Expression and Promoter Methylation Pro- files of Critical Genes Involved in Lung Cancer in NL-20 Lung Cells in Vitro: Short-Term and Chronic Exposure,” International Journal of Toxicology, Vol. 32, No. 1, 2013, pp. 23-31. doi:10.1177/1091581812465902 [84] D. Volanis, et al., “Molecular Mechanisms in Urinary Bladder Carcinogenesis,” Journal of Balkan Union of On- cology, Vol. 16, No. 4, 2011, pp. 589-601. [85] M. Brait, et al., “Genome-Wide Methylation Profiling and the PI3K-AKT Pathway Analysis Associated with Smok- ing in Urothelial Cell Carcinoma,” Cell Cycle, Vol. 12, No. 7, 2013, pp. 1058-1070. doi:10.4161/cc.24050 [86] D. Limsui, et al., “Cigarette Smoking and Colorectal Cancer Risk by Molecularly Defined Subtypes,” Journal of the National Cancer Institute, Vol. 102, No. 14, 2010, pp. 1012-1022. doi:10.1093/jnci/djq201 [87] E. S. Wan, et al., “Cigarette Smoking Behaviors and Time Since Quitting Are Associated with Differential DNA Methylation across the Human Genome,” Human Mole- cular Genetics, Vol. 21, No. 13, 2012, pp. 3073-3082. doi:10.1093/hmg/dds135 [88] H. Yao and I. Rahman, “Role of Histone Deacetylase 2 in Epigenetics and Cellular Senescence: Implications in Lung Inflammaging and COPD,” American Journal of Physiology—Lung Cellular and Molecular Physiology, Vol. 303, No. 7, 2012, pp. L557-L566. doi:10.1152/ajplung.00175.2012 [89] M. Stapleton, et al., “Smoking and Asthma,” The Journal of the American Board of Family Medicine, Vol. 24, No. 3, 2011, pp. 313-322. doi:10.3122/jabfm.2011.03.100180 [90] D. Adenuga, et al., “Histone Deacetylase 2 Is Phos- phorylated, Ubiquitinated, and Degraded by Cigarette Smoke,” American Journal of Respiratory Cell and Mole- cular Biology, Vol. 40, No. 4, 2009, pp. 464-473. doi:10.1165/rcmb.2008-0255OC [91] G. O. Osoata, et al., “Nitration of Distinct Tyrosine Re- sidues Causes Inactivation of Histone Deacetylase 2,” Biochemical and Biophysical Research Communications, Vol. 384, No. 3, 2009, pp. 366-371. doi:10.1016/j.bbrc.2009.04.128 [92] T. Toyooka and Y. Ibuki, “Cigarette Sidestream Smoke Induces Phosphorylated Histone H2AX,” Mutation Re- search, Vol. 676, No. 1-2, 2009, pp. 34-40. doi:10.1016/j.mrgentox.2009.03.002 [93] B. S. Kim, et al., “Xanthine Oxidoreductase Is a Critical Mediator of Cigarette Smoke-Induced Endothelial Cell DNA Damage and Apoptosis,” Free Radical Biology & Medicine, Vol. 60, 2013, pp. 336-346. doi:10.1016/j.freeradbiomed.2013.01.023 [94] I. K. Sundar, et al., “Mitogen- and Stress-Activated Ki- nase 1 (MSK1) Regulates Cigarette Smoke-Induced His- tone Modifications on NF-KappaB-Dependent Genes,” PLoS ONE, Vol. 7, No. 2, 2012, Article ID: e31378. doi:10.1371/journal.pone.0031378 [95] S. Chung, et al., “NF-KappaB Inducing Kinase, NIK Me- diates Cigarette Smoke/TNFalpha-Induced Histone Acety- lation and Inflammation through Differential Activation of IKKs,” PLoS ONE, Vol. 6, No. 8, 2011, Article ID: e23488. doi:10.1371/journal.pone.0023488 [96] M. Li, et al., “Effect of Erythromycin on Cigarette- Induced Histone Deacetylase Protein Expression and Nu- clear Factor-KappaB Activity in Human Macrophages in Vitro,” International Immunopharmacology, Vol. 12, No. 4, 2012, pp. 643-650. doi:10.1016/j.intimp.2011.12.022 [97] K. Takahashi, et al., “Cigarette Smoking Substantially Alters Plasma MicroRNA Profiles in Healthy Subjects,” Toxicology and Applied Pharmacology, 2013. (in Press) doi:10.1016/j.taap.2013.05.018 [98] F. Schembri, et al., “MicroRNAs as Modulators of Smo- king-Induced Gene Expression Changes in Human Air- way Epithelium,” Proceedings of the National Academy of Sciences of the United States of America, Vol. 106, No. 7, 2009, pp. 2319-2324. doi:10.1073/pnas.0806383106 [99] J. W. Graff, et al., “Cigarette Smoking Decreases Global MicroRNA Expression in Human Alveolar Macro-pha- ges,” PLoS ONE, Vol. 7, No. 8, 2012, Article ID: e44066. doi:10.1371/journal.pone.0044066 [100] S. Xi, et al., “Cigarette Smoke Induces C/EBP-Beta- Mediated Activation of miR-31 in Normal Human Res- Copyright © 2013 SciRes. JEP
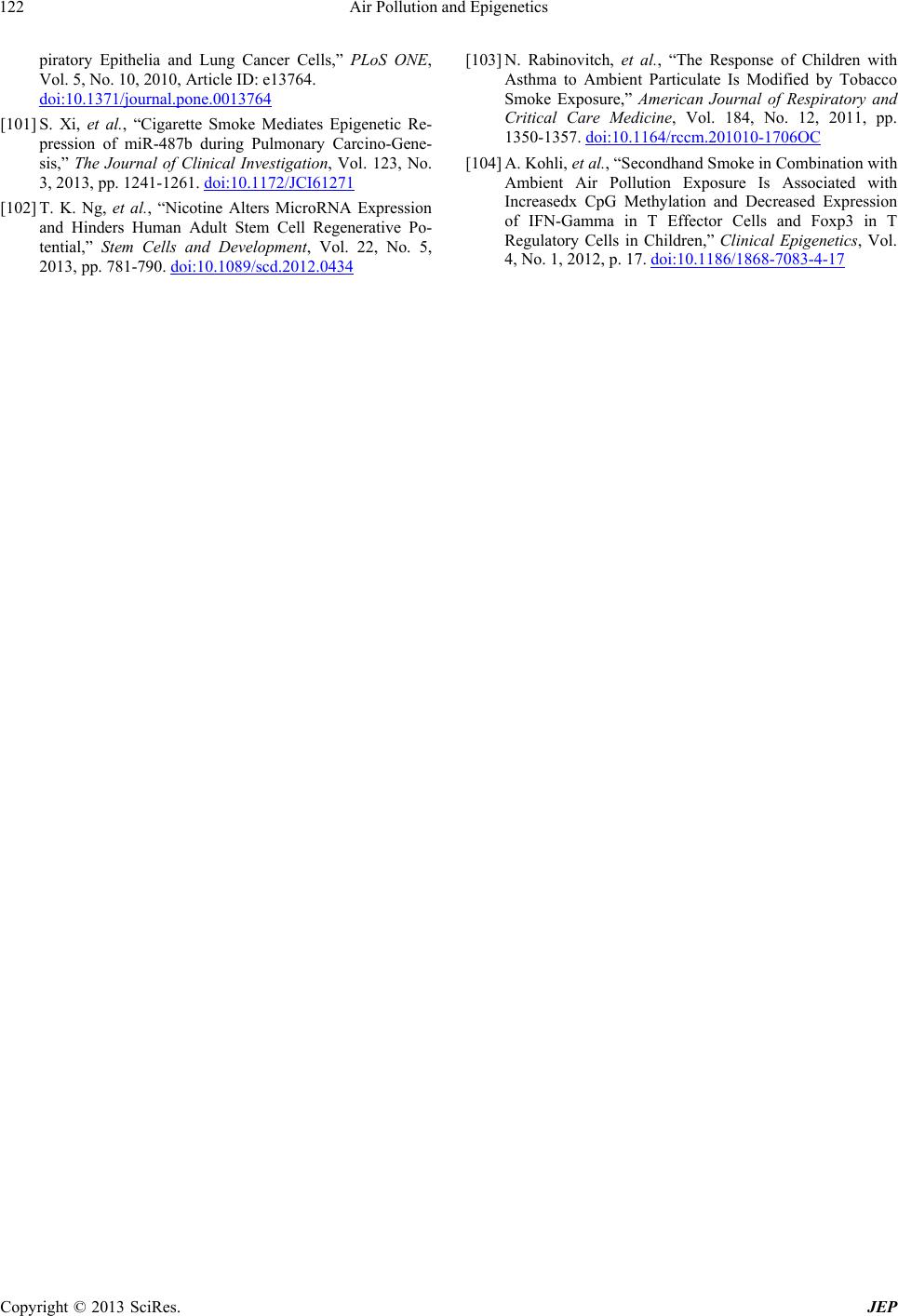 Air Pollution and Epigenetics Copyright © 2013 SciRes. JEP 122 piratory Epithelia and Lung Cancer Cells,” PLoS ONE, Vol. 5, No. 10, 2010, Article ID: e13764. doi:10.1371/journal.pone.0013764 [101] S. Xi, et al., “Cigarette Smoke Mediates Epigenetic Re- pression of miR-487b during Pulmonary Carcino-Gene- sis,” The Journal of Clinical Investigation, Vol. 123, No. 3, 2013, pp. 1241-1261. doi:10.1172/JCI61271 [102] T. K. Ng, et al., “Nicotine Alters MicroRNA Expression and Hinders Human Adult Stem Cell Regenerative Po- tential,” Stem Cells and Development, Vol. 22, No. 5, 2013, pp. 781-790. doi:10.1089/scd.2012.0434 [103] N. Rabinovitch, et al., “The Response of Children with Asthma to Ambient Particulate Is Modified by Tobacco Smoke Exposure,” American Journal of Respiratory and Critical Care Medicine, Vol. 184, No. 12, 2011, pp. 1350-1357. doi:10.1164/rccm.201010-1706OC [104] A. Kohli, et al., “Secondhand Smoke in Combination with Ambient Air Pollution Exposure Is Associated with Increasedx CpG Methylation and Decreased Expression of IFN-Gamma in T Effector Cells and Foxp3 in T Regulatory Cells in Children,” Clinical Epigenetics, Vol. 4, No. 1, 2012, p. 17. doi:10.1186/1868-7083-4-17
|