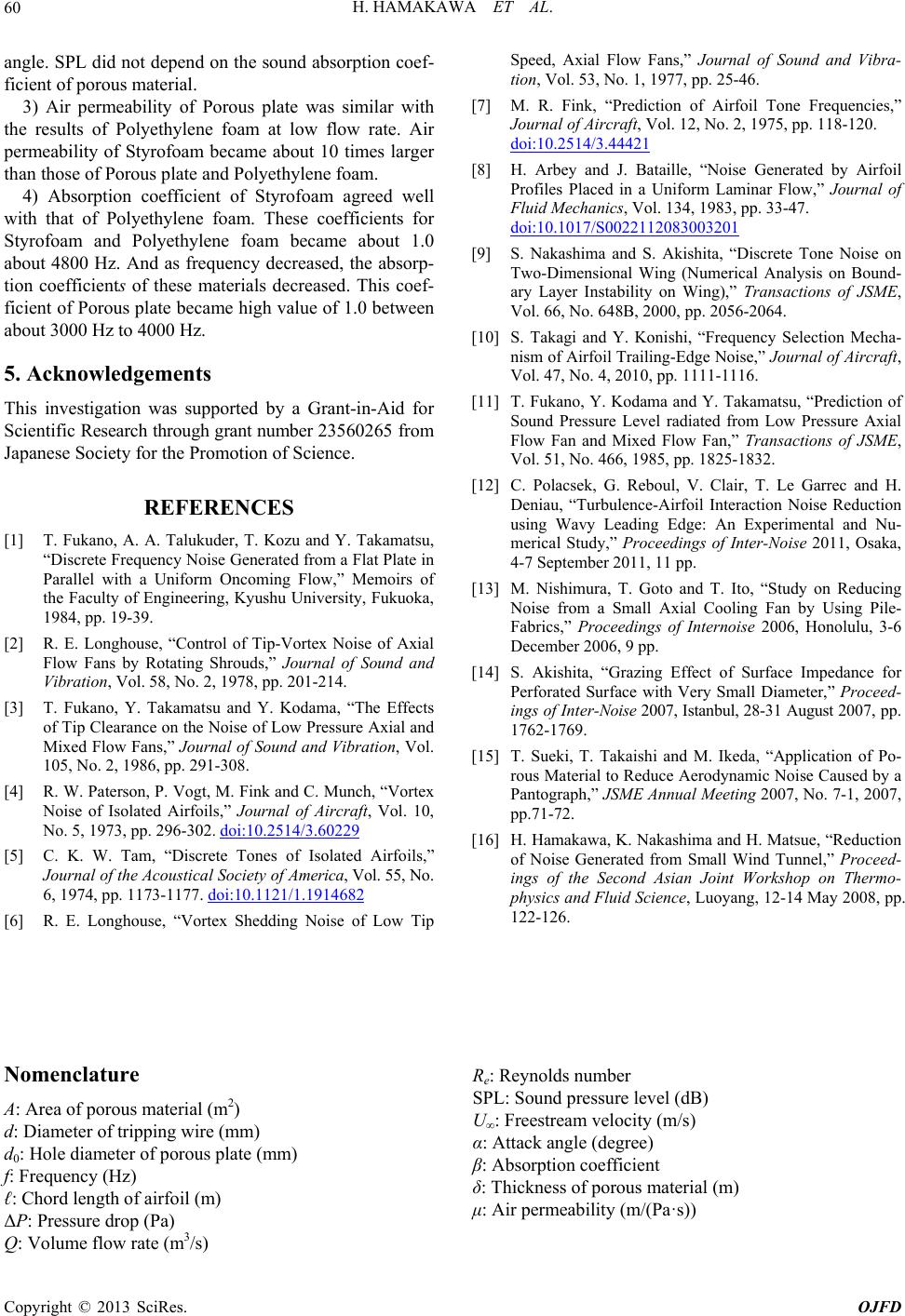
H. HAMAKAWA ET AL.
Copyright © 2013 SciRes. OJFD
60
on c
fic
cy decreased, the ab
tio
ol of Tip-Vortex Noise of Axial
Flow Fans by Rotating Shrouds,” Journal of Sound and
978, pp. 201-214.
[4] R. W. Paterson, P. Vogt, M. Fink and C. Munch, “Vortex
Noise of Isolatf Aircraft, Vol
No. 5, 1973, p3.60229
Speed, Axial Flow Fans,” Journal of Sound and Vibra-
tion, Vol. 53, No. 1, 1977, pp. 25-46.
[7] M. R. Fink, “Prediction of Airfoil Tone Freq
angle. SPL did not depend on the sound absorptioef-
ient of porous material.
3) Air permeability of Porous plate was similar with
the results of Polyethylene foam at low flow rate. Air
permeability of Styrofoam became about 10 times larger
than those of Porous plate and Polyethylene foam.
4) Absorption coefficient of Styrofoam agreed well
with that of Polyethylene foam. These coefficients for
Styrofoam and Polyethylene foam became about 1.0
about 4800 Hz. And as frequen
uencies,”
Journal of Aircraft, Vol. 12, No. 2, 1975, pp. 118-120.
doi:10.2514/3.44421
[8] H. Arbey and J. Bataille, “Noise Generated by Airfoil
Profiles Placed in a Uniform Laminar Flow,” Journal of
Fluid Mechanics, Vol. 134, 1983, pp. 33-47.
doi:10.1017/S0022112083003201
[9] S. Nakashima and S. Akishita, “Discrete Tone Noise on
Two-Dimensional Wing (Numerical Analysis on Bound
ary Layer Instability
sorp-
n coefficients of these materials decreased. This coef-
ficient of Porous plate became high value of 1.0 between
about 3000 Hz to 4000 Hz.
5. Acknowledgements
This investigation was supported by a Grant-in-Aid for
Scientific Research through grant number 23560265 from
Japanese Society for the Promotion of Science.
REFERENCES
[1] T. Fukano, A. A. Talukuder, T. Kozu and Y. Takamatsu,
“Discrete Frequency Noise Generated from a Flat Plate in
Parallel with a Uniform Oncoming Flow,” Memoirs of
the Faculty of Engineering, Kyushu University, Fukuoka,
1984, pp. 19-39.
[2] R. E. Longhouse, “Contr
Vibration, Vol. 58, No. 2, 1
[3] T. Fukano, Y. Takamatsu and Y. Kodama, “The Effects
of Tip Clearance on the Noise of Low Pressure Axial and
Mixed Flow Fans,” Journal of Sound and Vibration, Vol.
105, No. 2, 1986, pp. 291-308.
ed Airfoils,” Journal o
p. 296-302.
. 10,
doi:10.2514/
[5] C. K. W. Tam, “Discrete Tones of Isolated Airfoils,”
Journal of the Acoustical Society of America, Vol. 55, No.
6, 1974, pp. 1173-1177. doi:10.1121/1.1914682
[6] R. E. Longhouse, “Vortex Shedding Noise of Low Tip
-
on Wing),” Transactions of JSME,
of Aircraft,
6.
rimental and Nu-
by Using Pile-
nbul, 28-31 August 2007, pp.
nual Meeting 2007, No. 7-1, 2007,
Second Asian Joint Workshop on Thermo-
: Area of porous material (m2)
: Diameter of tripping wire (mm)
0: Hole diameter of porous plate (mm)
Frequency (Hz)
Chord length of airfoil (m
Pa)
e (m3/s)
mber
SPL: Sound pressure level (dB)
U∞: Freestream velocity (m/s)
α: Attack angle (degree)
β: Absorption coefficient
δ: Thickness of porous material (m)
μ: Air permeability (m/(Pa·s))
Vol. 66, No. 648B, 2000, pp. 2056-2064.
[10] S. Takagi and Y. Konishi, “Frequency Selection Mecha-
nism of Airfoil Trailing-Edge Noise,” Journal
Vol. 47, No. 4, 2010, pp. 1111-111
[11] T. Fukano, Y. Kodama and Y. Takamatsu, “Prediction of
Sound Pressure Level radiated from Low Pressure Axial
Flow Fan and Mixed Flow Fan,” Transactions of JSME,
Vol. 51, No. 466, 1985, pp. 1825-1832.
[12] C. Polacsek, G. Reboul, V. Clair, T. Le Garrec and H.
Deniau, “Turbulence-Airfoil Interaction Noise Reduction
using Wavy Leading Edge: An Expe
merical Study,” Proceedings of Inter-Noise 2011, Osaka,
4-7 September 2011, 11 pp.
[13] M. Nishimura, T. Goto and T. Ito, “Study on Reducing
Noise from a Small Axial Cooling Fan
Fabrics,” Proceedings of Internoise 2006, Honolulu, 3-6
December 2006, 9 pp.
[14] S. Akishita, “Grazing Effect of Surface Impedance for
Perforated Surface with Very Small Diameter,” Proceed-
ings of Inter-Noise 2007, Ista
1762-1769.
[15] T. Sueki, T. Takaishi and M. Ikeda, “Application of Po-
rous Material to Reduce Aerodynamic Noise Caused by a
Pantograph,” JSME An
pp.71-72.
[16] H. Hamakawa, K. Nakashima and H. Matsue, “Reduction
of Noise Generated from Small Wind Tunnel,” Proceed-
ings of the
physics and Fluid Science, Luoyang, 12-14 May 2008, pp.
122-126.
Nomenclature Re: Reynolds nu
A
d
d
f:
ℓ:)
ΔP: Pressure drop (
Q: Volume flow rat