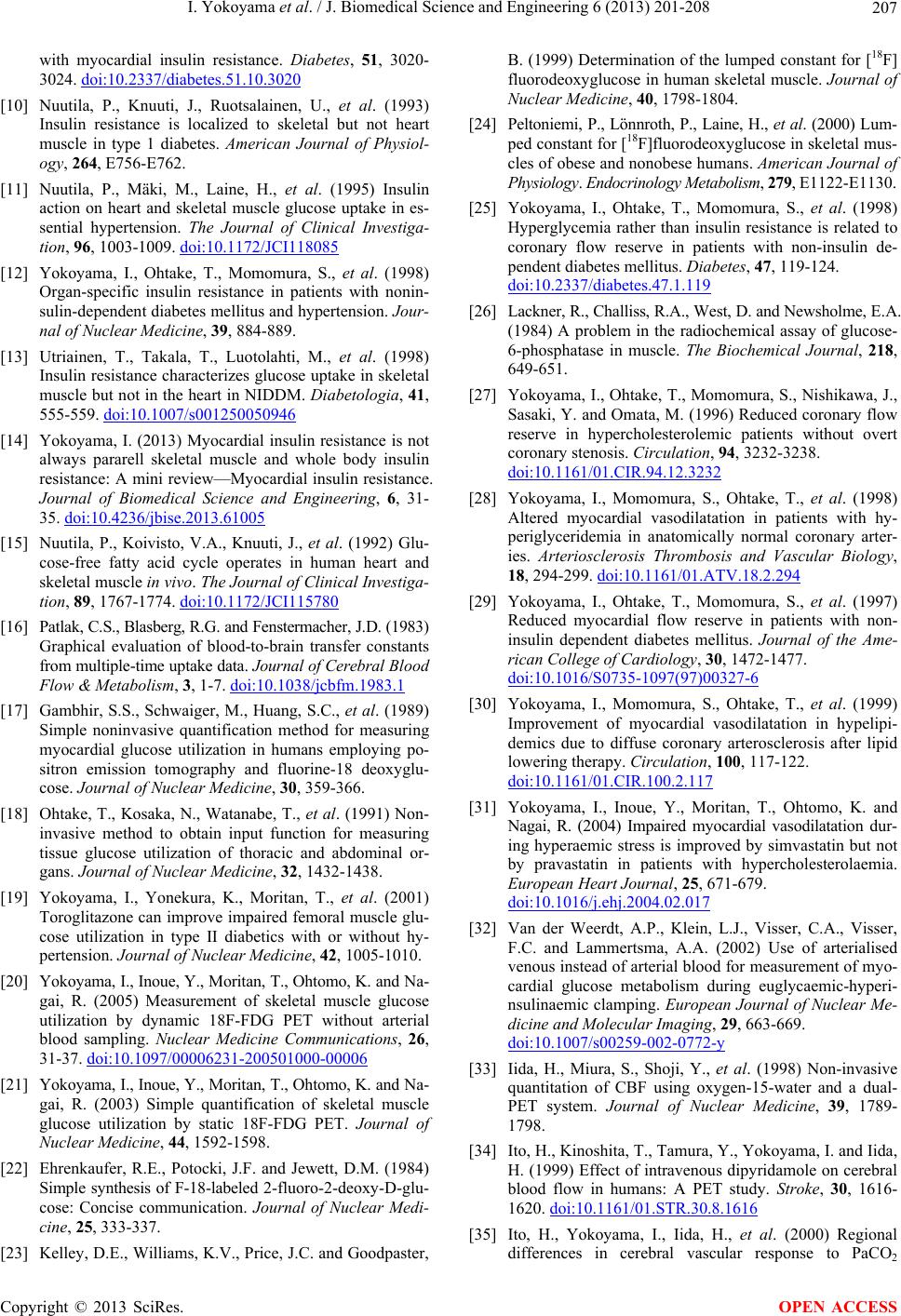
I. Yokoyama et al. / J. Biomedical Science and Engineering 6 (2013) 201-208 207
with myocardial insulin resistance. Diabetes, 51, 3020-
3024. doi:10.2337/diabetes.51.10.3020
[10] Nuutila, P., Knuuti, J., Ruotsalainen, U., et al. (1993)
Insulin resistance is localized to skeletal but not heart
muscle in type 1 diabetes. American Journal of Physiol-
ogy, 264, E756-E762.
[11] Nuutila, P., Mäki, M., Laine, H., et al. (1995) Insulin
action on heart and skeletal muscle glucose uptake in es-
sential hypertension. The Journal of Clinical Investiga-
tion, 96, 1003-1009. doi:10.1172/JCI118085
[12] Yokoyama, I., Ohtake, T., Momomura, S., et al. (1998)
Organ-specific insulin resistance in patients with nonin-
sulin-dependent diabetes mellitus and hypertension. Jour-
nal of Nuclear Medicine, 39, 884-889.
[13] Utriainen, T., Takala, T., Luotolahti, M., et al. (1998)
Insulin resistance characterizes glucose uptake in skeletal
muscle but not in the heart in NIDDM. Diabetologia, 41,
555-559. doi:10.1007/s001250050946
[14] Yokoyama, I. (2013) Myocardial insulin resistance is not
always pararell skeletal muscle and whole body insulin
resistance: A mini review—Myocardial insulin resistance.
Journal of Biomedical Science and Engineering, 6, 31-
35. doi:10.4236/jbise.2013.61005
[15] Nuutila, P., Koivisto, V.A., Knuuti, J., et al. (1992) Glu-
cose-free fatty acid cycle operates in human heart and
skeletal muscle in vivo. The Journal of Clinical Investiga-
tion, 89, 1767-1774. doi:10.1172/JCI115780
[16] Patlak, C.S., Blasberg, R.G. and Fenstermacher, J.D. (1983)
Graphical evaluation of blood-to-brain transfer constants
from multiple-time uptake data. Journal of Cerebral Blood
Flow & Metabolism, 3, 1-7. doi:10.1038/jcbfm.1983.1
[17] Gambhir, S.S., Schwaiger, M., Huang, S.C., et al. (1989)
Simple noninvasive quantification method for measuring
myocardial glucose utilization in humans employing po-
sitron emission tomography and fluorine-18 deoxyglu-
cose. Journal of Nuclear Medicine, 30, 359-366.
[18] Ohtake, T., Kosaka, N., Watanabe, T., et al . (1991) Non-
invasive method to obtain input function for measuring
tissue glucose utilization of thoracic and abdominal or-
gans. Journal of Nuclear Medicine, 32, 1432-1438.
[19] Yokoyama, I., Yonekura, K., Moritan, T., et al. (2001)
Toroglitazone can improve impaired femoral muscle glu-
cose utilization in type II diabetics with or without hy-
pertension. Journal of Nuclear Medicine, 42, 1005-1010.
[20] Yokoyama, I., Inoue, Y., Moritan, T., Ohtomo, K. and Na-
gai, R. (2005) Measurement of skeletal muscle glucose
utilization by dynamic 18F-FDG PET without arterial
blood sampling. Nuclear Medicine Communications, 26,
31-37. doi:10.1097/00006231-200501000-00006
[21] Yokoyama, I., Inoue, Y., Moritan, T., Ohtomo, K. and Na-
gai, R. (2003) Simple quantification of skeletal muscle
glucose utilization by static 18F-FDG PET. Journal of
Nuclear Medicine, 44, 1592-1598.
[22] Ehrenkaufer, R.E., Potocki, J.F. and Jewett, D.M. (1984)
Simple synthesis of F-18-labeled 2-fluoro-2-deoxy-D-glu-
cose: Concise communication. Journal of Nuclear Medi-
cine, 25, 333-337.
[23] Kelley, D.E., Williams, K.V., Price, J.C. and Goodpaster,
B. (1999) Determination of the lumped constant for [18F]
fluorodeoxyglucose in human skeletal muscle. Journal of
Nuclear Medicine, 40, 1798-1804.
[24] Peltoniemi, P., Lönnroth, P., Laine, H., et al. (2000) Lum-
ped constant for [18F]fluorodeoxyglucose in skeletal mus-
cles of obese and nonobese humans. American Journal of
Physiology. Endocrinology M etabo lism, 279, E1122-E1130.
[25] Yokoyama, I., Ohtake, T., Momomura, S., et al. (1998)
Hyperglycemia rather than insulin resistance is related to
coronary flow reserve in patients with non-insulin de-
pendent diabetes mellitus. Diabetes, 47, 119-124.
doi:10.2337/diabetes.47.1.119
[26] Lackner, R., Challiss, R.A., West, D. and Newsholme, E.A.
(1984) A problem in the radiochemical assay of glucose-
6-phosphatase in muscle. The Biochemical Journal, 218,
649-651.
[27] Yokoyama, I., Ohtake, T., Momomura, S., Nishikawa, J.,
Sasaki, Y. and Omata, M. (1996) Reduced coronary flow
reserve in hypercholesterolemic patients without overt
coronary stenosis. Circulation, 94, 3232-3238.
doi:10.1161/01.CIR.94.12.3232
[28] Yokoyama, I., Momomura, S., Ohtake, T., et al. (1998)
Altered myocardial vasodilatation in patients with hy-
periglyceridemia in anatomically normal coronary arter-
ies. Arteriosclerosis Thrombosis and Vascular Biology,
18, 294-299. doi:10.1161/01.ATV.18.2.294
[29] Yokoyama, I., Ohtake, T., Momomura, S., et al. (1997)
Reduced myocardial flow reserve in patients with non-
insulin dependent diabetes mellitus. Journal of the Ame-
rican College of Cardiology, 30, 1472-1477.
doi:10.1016/S0735-1097(97)00327-6
[30] Yokoyama, I., Momomura, S., Ohtake, T., et al. (1999)
Improvement of myocardial vasodilatation in hypelipi-
demics due to diffuse coronary arterosclerosis after lipid
lowering therapy. Circulation, 100, 117-122.
doi:10.1161/01.CIR.100.2.117
[31] Yokoyama, I., Inoue, Y., Moritan, T., Ohtomo, K. and
Nagai, R. (2004) Impaired myocardial vasodilatation dur-
ing hyperaemic stress is improved by simvastatin but not
by pravastatin in patients with hypercholesterolaemia.
European Heart Journal, 25, 671-679.
doi:10.1016/j.ehj.2004.02.017
[32] Van der Weerdt, A.P., Klein, L.J., Visser, C.A., Visser,
F.C. and Lammertsma, A.A. (2002) Use of arterialised
venous instead of arterial blood for measurement of myo-
cardial glucose metabolism during euglycaemic-hyperi-
nsulinaemic clamping. European Journal of Nuclear Me-
dicine and Molecular Imaging, 29, 663-669.
doi:10.1007/s00259-002-0772-y
[33] Iida, H., Miura, S., Shoji, Y., et al. (1998) Non-invasive
quantitation of CBF using oxygen-15-water and a dual-
PET system. Journal of Nuclear Medicine, 39, 1789-
1798.
[34] Ito, H., Kinoshita, T., Tamura, Y., Yokoyama, I. and Iida,
H. (1999) Effect of intravenous dipyridamole on cerebral
blood flow in humans: A PET study. Stroke, 30, 1616-
1620. doi:10.1161/01.STR.30.8.1616
[35] Ito, H., Yokoyama, I., Iida, H., et al. (2000) Regional
differences in cerebral vascular response to PaCO2
Copyright © 2013 SciRes. OPEN ACCESS