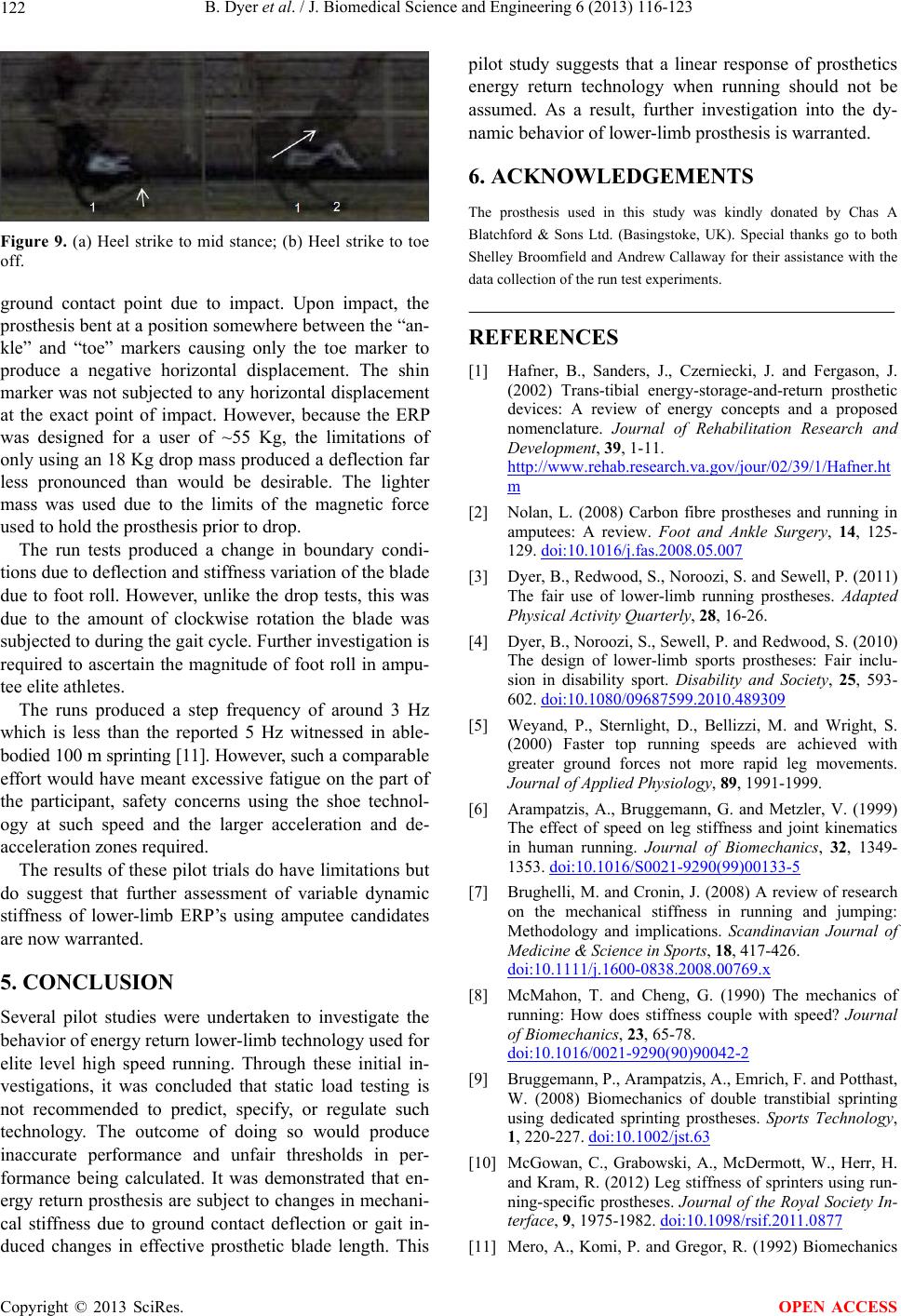
B. Dyer et al. / J. Biomedical Science and Engineering 6 (2013) 116-123
122
Figure 9. (a) Heel strike to mid stance; (b) Heel strike to toe
off.
ground contact point due to impact. Upon impact, the
prosthesis bent at a position somewhere between the “an-
kle” and “toe” markers causing only the toe marker to
produce a negative horizontal displacement. The shin
marker was not subjected to any horizontal displacement
at the exact point of impact. However, because the ERP
was designed for a user of ~55 Kg, the limitations of
only using an 18 Kg drop mass produced a deflection far
less pronounced than would be desirable. The lighter
mass was used due to the limits of the magnetic force
used to hold the prosthesis pr ior to drop.
The run tests produced a change in boundary condi-
tions due to deflection and stiffness variation of the blade
due to foot roll. However, unlike the drop tests, this was
due to the amount of clockwise rotation the blade was
subjected to during th e gait cycle. Further investigation is
required to ascertain the magnitude of foot roll in ampu-
tee elite athletes.
The runs produced a step frequency of around 3 Hz
which is less than the reported 5 Hz witnessed in able-
bodied 100 m sprinting [11]. However, such a co mpa r ab le
effort would have meant excessive fatigue on the part of
the participant, safety concerns using the shoe technol-
ogy at such speed and the larger acceleration and de-
acceleration zones required.
The results of these p ilot trials do have limitations bu t
do suggest that further assessment of variable dynamic
stiffness of lower-limb ERP’s using amputee candidates
are now warranted.
5. CONCLUSION
Several pilot studies were undertaken to investigate the
behavior of energy return lower-limb technology used for
elite level high speed running. Through these initial in-
vestigations, it was concluded that static load testing is
not recommended to predict, specify, or regulate such
technology. The outcome of doing so would produce
inaccurate performance and unfair thresholds in per-
formance being calculated. It was demonstrated that en-
ergy return prosthesis are subject to changes in mechani-
cal stiffness due to ground contact deflection or gait in-
duced changes in effective prosthetic blade length. This
pilot study suggests that a linear response of prosthetics
energy return technology when running should not be
assumed. As a result, further investigation into the dy-
namic behavior of lower-limb prosthesis is warranted.
6. ACKNOWLEDGEMENTS
The prosthesis used in this study was kindly donated by Chas A
Blatchford & Sons Ltd. (Basingstoke, UK). Special thanks go to both
Shelley Broomfield and Andrew Callaway for their assistance with the
data collection of the run t est experiments.
REFERENCES
[1] Hafner, B., Sanders, J., Czerniecki, J. and Fergason, J.
(2002) Trans-tibial energy-storage-and-return prosthetic
devices: A review of energy concepts and a proposed
nomenclature. Journal of Rehabilitation Research and
Development, 39, 1-11.
http://www.rehab.research.va.gov/jour/02/39/1/Hafner.ht
m
[2] Nolan, L. (2008) Carbon fibre prostheses and running in
amputees: A review. Foot and Ankle Surgery, 14, 125-
129. doi:10.1016/j.fas.2008.05.007
[3] Dyer, B., Redwood, S., Noroozi, S. and Sewell, P. (2011)
The fair use of lower-limb running prostheses. Adapted
Physical Activity Quarterly, 28, 16-26.
[4] Dyer, B., Noroozi, S., Sewell, P. and Redwood, S. (2010)
The design of lower-limb sports prostheses: Fair inclu-
sion in disability sport. Disability and Society, 25, 593-
602. doi:10.1080/09687599.2010.489309
[5] Weyand, P., Sternlight, D., Bellizzi, M. and Wright, S.
(2000) Faster top running speeds are achieved with
greater ground forces not more rapid leg movements.
Journal of Applied Physiology, 89, 1991-1999.
[6] Arampatzis, A., Bruggemann, G. and Metzler, V. (1999)
The effect of speed on leg stiffness and joint kinematics
in human running. Journal of Biomechanics, 32, 1349-
1353. doi:10.1016/S0021-9290(99)00133-5
[7] Brughelli, M. and Cronin, J. (2008) A review of research
on the mechanical stiffness in running and jumping:
Methodology and implications. Scandinavian Journal of
Medicine & Science in Sports, 18, 417-426.
doi:10.1111/j.1600-0838.2008.00769.x
[8] McMahon, T. and Cheng, G. (1990) The mechanics of
running: How does stiffness couple with speed? Journal
of Biomechanics, 23, 65-78.
doi:10.1016/0021-9290(90)90042-2
[9] Bruggemann, P., Arampatzis, A., Emrich, F. and Potthast,
W. (2008) Biomechanics of double transtibial sprinting
using dedicated sprinting prostheses. Sports Technology,
1, 220-227. doi:10.1002/jst.63
[10] McGowan, C., Grabowski, A., McDermott, W., Herr, H.
and Kram, R. (2012) Leg stiffness of sprinters using run-
ning-specific prostheses. Journal of the Royal Society In-
terface, 9, 1975-1982. doi:10.1098/rsif.2011.0877
[11] Mero, A., Komi, P. and Gregor, R. (1992) Biomechanics
Copyright © 2013 SciRes. OPEN ACCESS