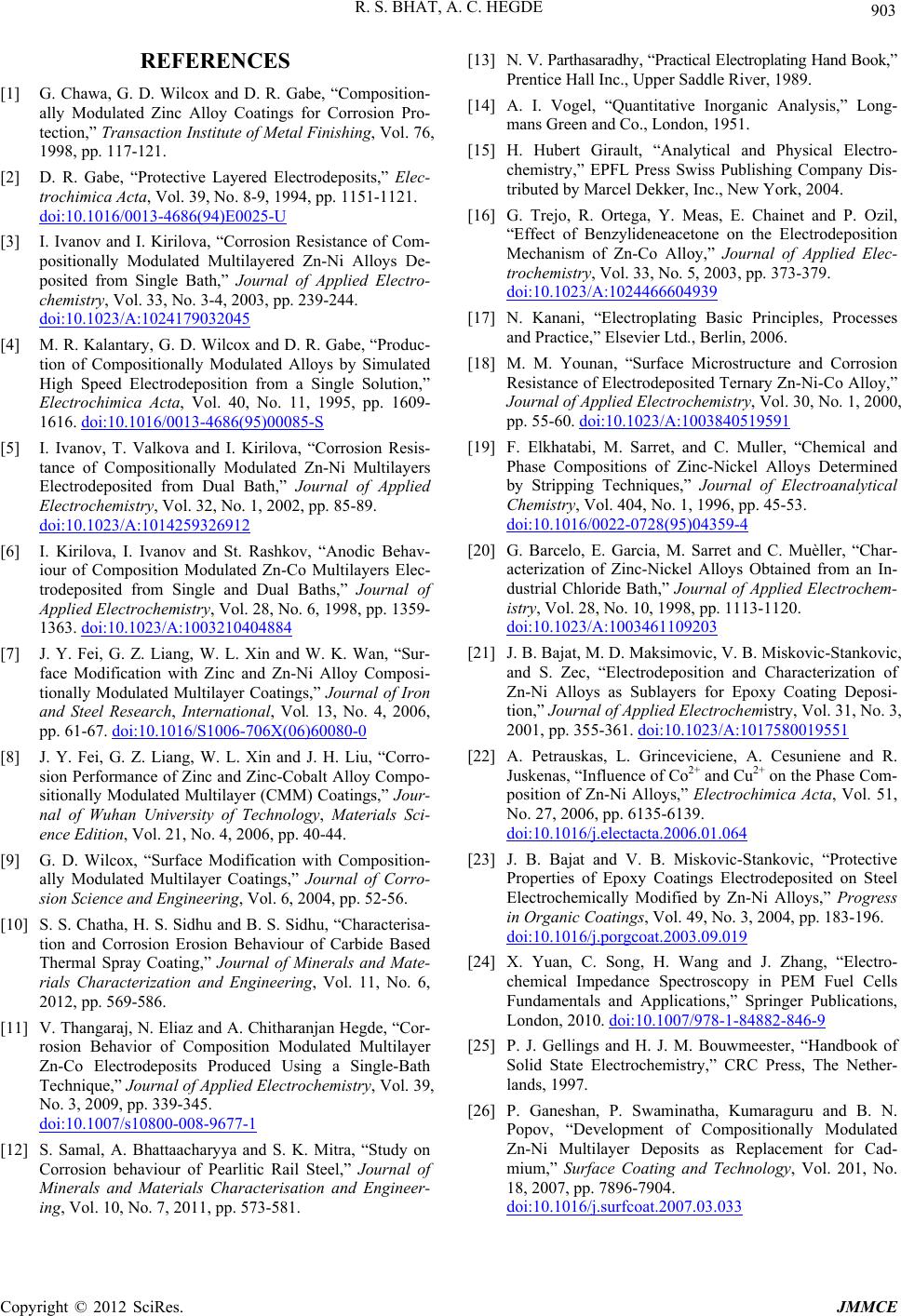
R. S. BHAT, A. C. HEGDE
Copyright © 2012 SciRes. JMMCE
903
REFERENCES
[1] G. Chawa, G. D. Wilcox and D. R. Gabe, “Composition-
ally Modulated Zinc Alloy Coatings for Corrosion Pro-
tection,” Transaction Institute of Metal Finishing, Vol. 76,
1998, pp. 117-121.
[2] D. R. Gabe, “Protective Layered Electrodeposits,” Elec-
trochimica Acta, Vol. 39, No. 8-9, 1994, pp. 1151-1121.
doi:10.1016/0013-4686(94)E0025-U
[3] I. Ivanov and I. Kirilova, “Corrosion Resistance of Com-
positionally Modulated Multilayered Zn-Ni Alloys De-
posited from Single Bath,” Journal of Applied Electro-
chemistry, Vol. 33, No. 3-4, 2003, pp. 239-244.
doi:10.1023/A:1024179032045
[4] M. R. Kalantary, G. D. Wilcox and D. R. Gabe, “Produc-
tion of Compositionally Modulated Alloys by Simulated
High Speed Electrodeposition from a Single Solution,”
Electrochimica Acta, Vol. 40, No. 11, 1995, pp. 1609-
1616. doi:10.1016/0013-4686(95)00085-S
[5] I. Ivanov, T. Valkova and I. Kirilova, “Corrosion Resis-
tance of Compositionally Modulated Zn-Ni Multilayers
Electrodeposited from Dual Bath,” Journal of Applied
Electrochemistry, Vol. 32, No. 1, 2002, pp. 85-89.
doi:10.1023/A:1014259326912
[6] I. Kirilova, I. Ivanov and St. Rashkov, “Anodic Behav-
iour of Composition Modulated Zn-Co Multilayers Elec-
trodeposited from Single and Dual Baths,” Journal of
Applied Electrochemistry, Vol. 28, No. 6, 1998, pp. 1359-
1363. doi:10.1023/A:1003210404884
[7] J. Y. Fei, G. Z. Liang, W. L. Xin and W. K. Wan, “Sur-
face Modification with Zinc and Zn-Ni Alloy Composi-
tionally Modulated Multilayer Coatings,” Journal of Iron
and Steel Research, International, Vol. 13, No. 4, 2006,
pp. 61-67. doi:10.1016/S1006-706X(06)60080-0
[8] J. Y. Fei, G. Z. Liang, W. L. Xin and J. H. Liu, “Corro-
sion Performance of Zinc and Zinc-Cobalt Alloy Compo-
sitionally Modulated Multilayer (CMM) Coatings,” Jour-
nal of Wuhan University of Technology, Materials Sci-
ence Edition, Vol. 21, No. 4, 2006, pp. 40-44.
[9] G. D. Wilcox, “Surface Modification with Composition-
ally Modulated Multilayer Coatings,” Journal of Corro-
sion Science and Engineering, Vol. 6, 2004, pp. 52-56.
[10] S. S. Chatha, H. S. Sidhu and B. S. Sidhu, “Characterisa-
tion and Corrosion Erosion Behaviour of Carbide Based
Thermal Spray Coating,” Journal of Minerals and Mate-
rials Characterization and Engineering, Vol. 11, No. 6,
2012, pp. 569-586.
[11] V. Thangaraj, N. Eliaz and A. Chitharanjan Hegde, “Cor-
rosion Behavior of Composition Modulated Multilayer
Zn-Co Electrodeposits Produced Using a Single-Bath
Technique,” Journal of Applied Electrochemistry, Vol. 39,
No. 3, 2009, pp. 339-345.
doi:10.1007/s10800-008-9677-1
[12] S. Samal, A. Bhattaacharyya and S. K. Mitra, “Study on
Corrosion behaviour of Pearlitic Rail Steel,” Journal of
Minerals and Materials Characterisation and Engineer-
ing, Vol. 10, No. 7, 2011, pp. 573-581.
[13] N. V. Parthasaradhy, “Practical Electroplating Hand Book,”
Prentice Hall Inc., Upper Saddle River, 1989.
[14] A. I. Vogel, “Quantitative Inorganic Analysis,” Long-
mans Green and Co., London, 1951.
[15] H. Hubert Girault, “Analytical and Physical Electro-
chemistry,” EPFL Press Swiss Publishing Company Dis-
tributed by Marcel Dekker, Inc., New York, 2004.
[16] G. Trejo, R. Ortega, Y. Meas, E. Chainet and P. Ozil,
“Effect of Benzylideneacetone on the Electrodeposition
Mechanism of Zn-Co Alloy,” Journal of Applied Elec-
trochemistry, Vol. 33, No. 5, 2003, pp. 373-379.
doi:10.1023/A:1024466604939
[17] N. Kanani, “Electroplating Basic Principles, Processes
and Practice,” Elsevier Ltd., Berlin, 2006.
[18] M. M. Younan, “Surface Microstructure and Corrosion
Resistance of Electrodeposited Ternary Zn-Ni-Co Alloy,”
Journal of Applied Electrochemistry, Vol. 30, No. 1, 2000,
pp. 55-60. doi:10.1023/A:1003840519591
[19] F. Elkhatabi, M. Sarret, and C. Muller, “Chemical and
Phase Compositions of Zinc-Nickel Alloys Determined
by Stripping Techniques,” Journal of Electroanalytical
Chemistry, Vol. 404, No. 1, 1996, pp. 45-53.
doi:10.1016/0022-0728(95)04359-4
[20] G. Barcelo, E. Garcia, M. Sarret and C. Muèller, “Char-
acterization of Zinc-Nickel Alloys Obtained from an In-
dustrial Chloride Bath,” Journal of Applied Electrochem-
istry, Vol. 28, No. 10, 1998, pp. 1113-1120.
doi:10.1023/A:1003461109203
[21] J. B. Bajat, M. D. Maksimovic, V. B. Miskovic-Stankovic,
and S. Zec, “Electrodeposition and Characterization of
Zn-Ni Alloys as Sublayers for Epoxy Coating Deposi-
tion,” Journal of Applied Electrochemistry, Vol. 31, No. 3,
2001, pp. 355-361. doi:10.1023/A:1017580019551
[22] A. Petrauskas, L. Grinceviciene, A. Cesuniene and R.
Juskenas, “Influence of Co2+ and Cu2+ on the Phase Com-
position of Zn-Ni Alloys,” Electrochimica Acta, Vol. 51,
No. 27, 2006, pp. 6135-6139.
doi:10.1016/j.electacta.2006.01.064
[23] J. B. Bajat and V. B. Miskovic-Stankovic, “Protective
Properties of Epoxy Coatings Electrodeposited on Steel
Electrochemically Modified by Zn-Ni Alloys,” Progress
in Organic Coatings, Vol. 49, No. 3, 2004, pp. 183-196.
doi:10.1016/j.porgcoat.2003.09.019
[24] X. Yuan, C. Song, H. Wang and J. Zhang, “Electro-
chemical Impedance Spectroscopy in PEM Fuel Cells
Fundamentals and Applications,” Springer Publications,
London, 2010. doi:10.1007/978-1-84882-846-9
[25] P. J. Gellings and H. J. M. Bouwmeester, “Handbook of
Solid State Electrochemistry,” CRC Press, The Nether-
lands, 1997.
[26] P. Ganeshan, P. Swaminatha, Kumaraguru and B. N.
Popov, “Development of Compositionally Modulated
Zn-Ni Multilayer Deposits as Replacement for Cad-
mium,” Surface Coating and Technology, Vol. 201, No.
18, 2007, pp. 7896-7904.
doi:10.1016/j.surfcoat.2007.03.033