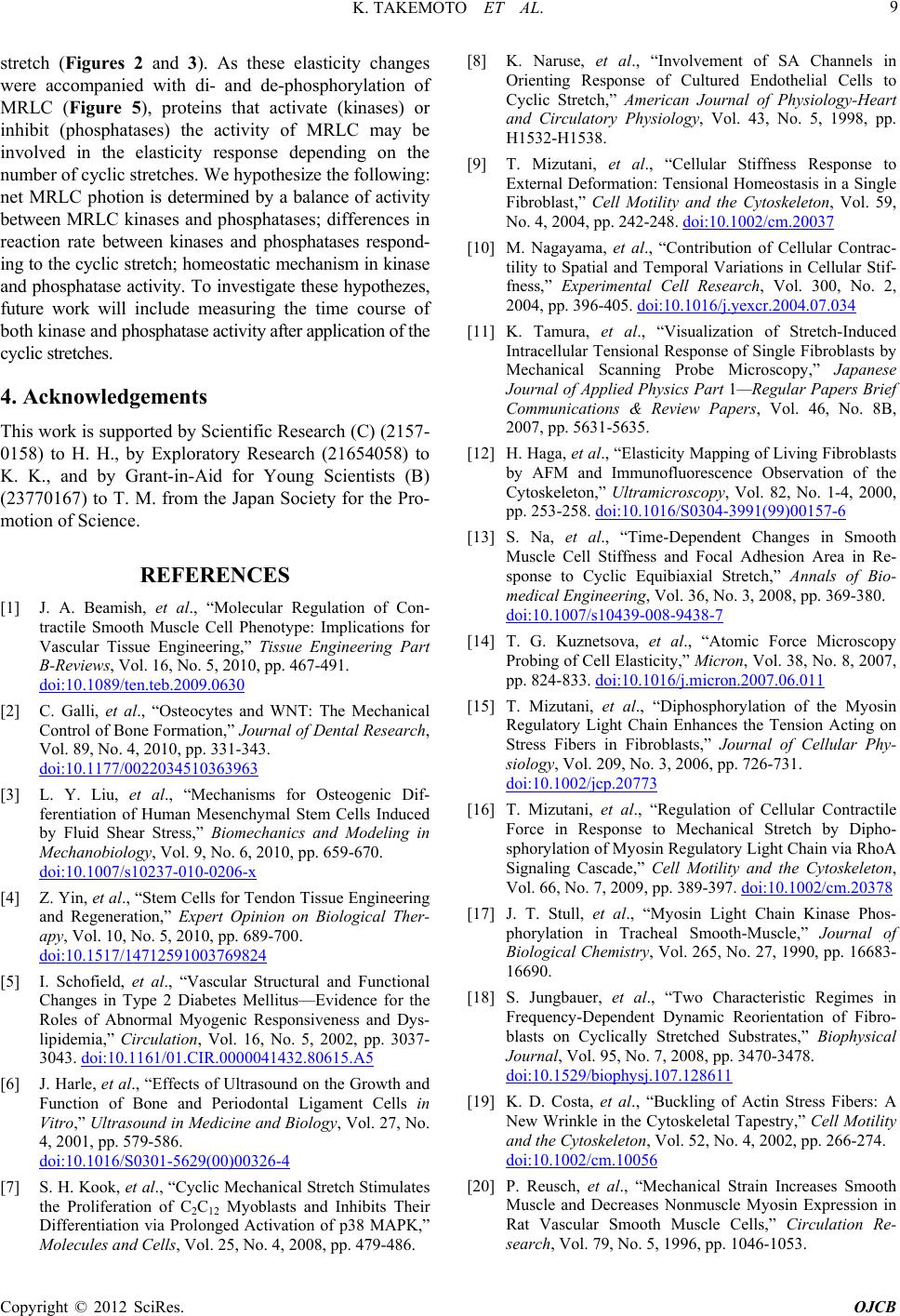
K. TAKEMOTO ET AL. 9
stretch (Figures 2 and 3). As these elasticity changes
were accompanied with di- and de-phosphorylation of
MRLC (Figure 5), proteins that activate (kinases) or
inhibit (phosphatases) the activity of MRLC may be
involved in the elasticity response depending on the
number of cyclic stretches. We hypothesize the following:
net MRLC photion is determined by a balance of activity
between MRLC kinases and phosphatases; differences in
reaction rate between kinases and phosphatases respond-
ing to the cyclic stretch; homeostatic mechanism in kinase
and phosphatase activity. To investigate these hypothezes,
future work will include measuring the time course of
both kinase and phosphatase activity after application of the
cyclic stretches.
4. Acknowledgements
This work is supported by Scientific Research (C) (2157-
0158) to H. H., by Exploratory Research (21654058) to
K. K., and by Grant-in-Aid for Young Scientists (B)
(23770167) to T. M. from the Japan Society for the Pro-
motion of Science.
REFERENCES
[1] J. A. Beamish, et al., “Molecular Regulation of Con-
tractile Smooth Muscle Cell Phenotype: Implications for
Vascular Tissue Engineering,” Tissue Engineering Part
B-Reviews, Vol. 16, No. 5, 2010, pp. 467-491.
doi:10.1089/ten.teb.2009.0630
[2] C. Galli, et al., “Osteocytes and WNT: The Mechanical
Control of Bone Formation,” Journal of Dental Research,
Vol. 89, No. 4, 2010, pp. 331-343.
doi:10.1177/0022034510363963
[3] L. Y. Liu, et al., “Mechanisms for Osteogenic Dif-
ferentiation of Human Mesenchymal Stem Cells Induced
by Fluid Shear Stress,” Biomechanics and Modeling in
Mechanobiology, Vol. 9, No. 6, 2010, pp. 659-670.
doi:10.1007/s10237-010-0206-x
[4] Z. Yin, et al., “Stem Cells for Tendon Tissue Engineering
and Regeneration,” Expert Opinion on Biological Ther-
apy, Vol. 10, No. 5, 2010, pp. 689-700.
doi:10.1517/14712591003769824
[5] I. Schofield, et al., “Vascular Structural and Functional
Changes in Type 2 Diabetes Mellitus—Evidence for the
Roles of Abnormal Myogenic Responsiveness and Dys-
lipidemia,” Circulation, Vol. 16, No. 5, 2002, pp. 3037-
3043. doi:10.1161/01.CIR.0000041432.80615.A5
[6] J. Harle, et al., “Effects of Ultrasound on the Growth and
Function of Bone and Periodontal Ligament Cells in
Vitro,” Ultrasound in Medicine and Biology, Vol. 27, No.
4, 2001, pp. 579-586.
doi:10.1016/S0301-5629(00)00326-4
[7] S. H. Kook, et al., “Cyclic Mechanical Stretch Stimulates
the Proliferation of C2C12 Myoblasts and Inhibits Their
Differentiation via Prolonged Activation of p38 MAPK,”
Molecules and Cells, Vol. 25, No. 4, 2008, pp. 479-486.
[8] K. Naruse, et al., “Involvement of SA Channels in
Orienting Response of Cultured Endothelial Cells to
Cyclic Stretch,” American Journal of Physiology-Heart
and Circulatory Physiology, Vol. 43, No. 5, 1998, pp.
H1532-H1538.
[9] T. Mizutani, et al., “Cellular Stiffness Response to
External Deformation: Tensional Homeostasis in a Single
Fibroblast,” Cell Motility and the Cytoskeleton, Vol. 59,
No. 4, 2004, pp. 242-248. doi:10.1002/cm.20037
[10] M. Nagayama, et al., “Contribution of Cellular Contrac-
tility to Spatial and Temporal Variations in Cellular Stif-
fness,” Experimental Cell Research, Vol. 300, No. 2,
2004, pp. 396-405. doi:10.1016/j.yexcr.2004.07.034
[11] K. Tamura, et al., “Visualization of Stretch-Induced
Intracellular Tensional Response of Single Fibroblasts by
Mechanical Scanning Probe Microscopy,” Japanese
Journal of Applied Physics Part 1—Regular Papers Brief
Communications & Review Papers, Vol. 46, No. 8B,
2007, pp. 5631-5635.
[12] H. Haga, et al., “Elasticity Mapping of Living Fibroblasts
by AFM and Immunofluorescence Observation of the
Cytoskeleton,” Ultramicroscopy, Vol. 82, No. 1-4, 2000,
pp. 253-258. doi:10.1016/S0304-3991(99)00157-6
[13] S. Na, et al., “Time-Dependent Changes in Smooth
Muscle Cell Stiffness and Focal Adhesion Area in Re-
sponse to Cyclic Equibiaxial Stretch,” Annals of Bio-
medical Engineering, Vol. 36, No. 3, 2008, pp. 369-380.
doi:10.1007/s10439-008-9438-7
[14] T. G. Kuznetsova, et al., “Atomic Force Microscopy
Probing of Cell Elasticity,” Micron, Vol. 38, No. 8, 2007,
pp. 824-833. doi:10.1016/j.micron.2007.06.011
[15] T. Mizutani, et al., “Diphosphorylation of the Myosin
Regulatory Light Chain Enhances the Tension Acting on
Stress Fibers in Fibroblasts,” Journal of Cellular Phy-
siology, Vol. 209, No. 3, 2006, pp. 726-731.
doi:10.1002/jcp.20773
[16] T. Mizutani, et al., “Regulation of Cellular Contractile
Force in Response to Mechanical Stretch by Dipho-
sphorylation of Myosin Regulatory Light Chain via RhoA
Signaling Cascade,” Cell Motility and the Cytoskeleton,
Vol. 66, No. 7, 2009, pp. 389-397. doi:10.1002/cm.20378
[17] J. T. Stull, et al., “Myosin Light Chain Kinase Phos-
phorylation in Tracheal Smooth-Muscle,” Journal of
Biological Chemistry, Vol. 265, No. 27, 1990, pp. 16683-
16690.
[18] S. Jungbauer, et al., “Two Characteristic Regimes in
Frequency-Dependent Dynamic Reorientation of Fibro-
blasts on Cyclically Stretched Substrates,” Biophysical
Journal, Vol. 95, No. 7, 2008, pp. 3470-3478.
doi:10.1529/biophysj.107.128611
[19] K. D. Costa, et al., “Buckling of Actin Stress Fibers: A
New Wrinkle in the Cytoskeletal Tapestry,” Cell Motility
and the Cytoskeleton, Vol. 52, No. 4, 2002, pp. 266-274.
doi:10.1002/cm.10056
[20] P. Reusch, et al., “Mechanical Strain Increases Smooth
Muscle and Decreases Nonmuscle Myosin Expression in
Rat Vascular Smooth Muscle Cells,” Circulation Re-
search, Vol. 79, No. 5, 1996, pp. 1046-1053.
Copyright © 2012 SciRes. OJCB