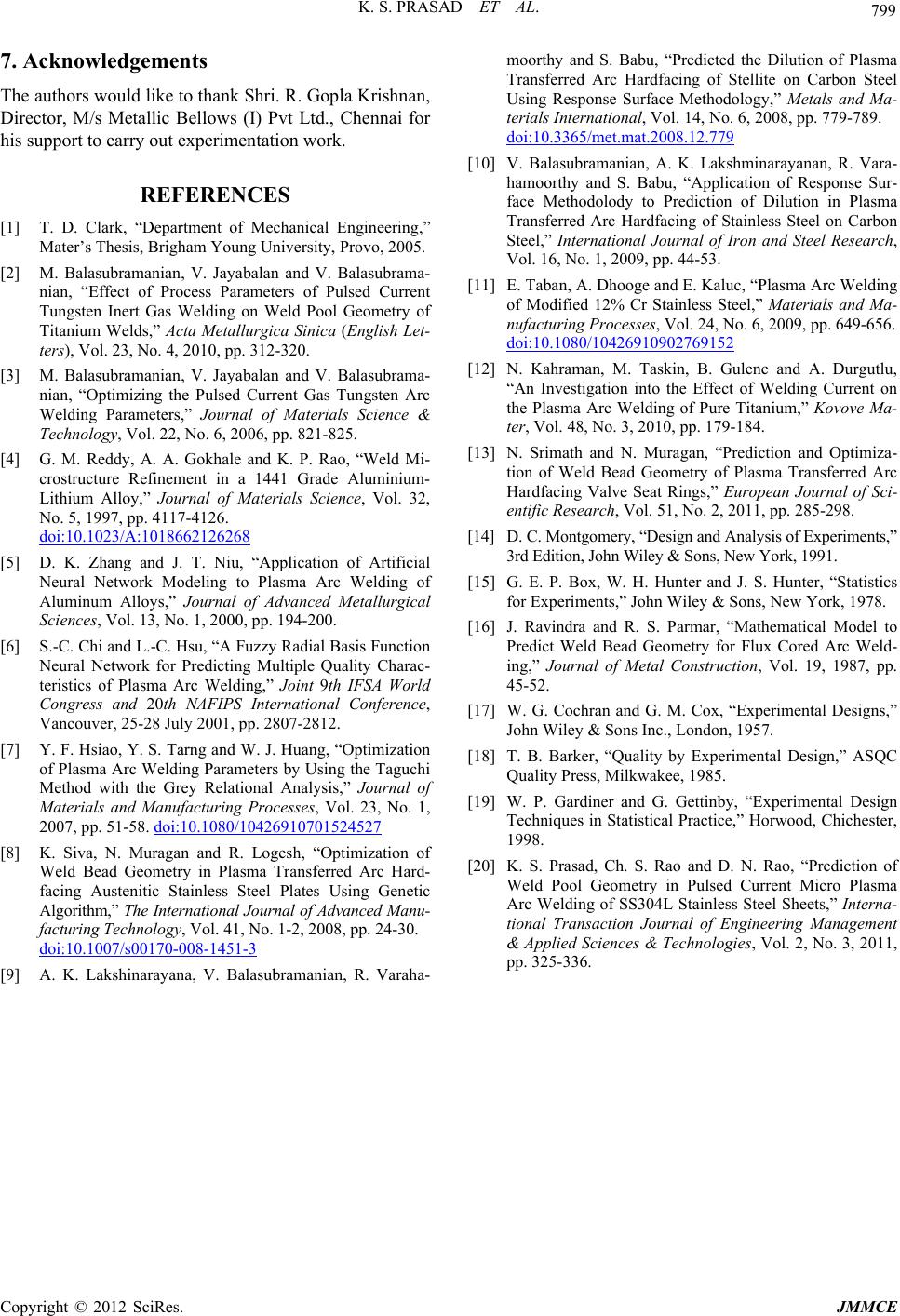
K. S. PRASAD ET AL. 799
7. Acknowledgements
The authors would like to thank Shri. R. Gopla Krishnan,
Director, M/s Metallic Bellows (I) Pvt Ltd., Chennai for
his support to carry out experimentation work.
REFERENCES
[1] T. D. Clark, “Department of Mechanical Engineering,”
Mater’s Thesis, Brigham Young University, Provo, 2005.
[2] M. Balasubramanian, V. Jayabalan and V. Balasubrama-
nian, “Effect of Process Parameters of Pulsed Current
Tungsten Inert Gas Welding on Weld Pool Geometry of
Titanium Welds,” Acta Metallurgica Sinica (English Let-
ters), Vol. 23, No. 4, 2010, pp. 312-320.
[3] M. Balasubramanian, V. Jayabalan and V. Balasubrama-
nian, “Optimizing the Pulsed Current Gas Tungsten Arc
Welding Parameters,” Journal of Materials Science &
Technology, Vol. 22, No. 6, 2006, pp. 821-825.
[4] G. M. Reddy, A. A. Gokhale and K. P. Rao, “Weld Mi-
crostructure Refinement in a 1441 Grade Aluminium-
Lithium Alloy,” Journal of Materials Science, Vol. 32,
No. 5, 1997, pp. 4117-4126.
doi:10.1023/A:1018662126268
[5] D. K. Zhang and J. T. Niu, “Application of Artificial
Neural Network Modeling to Plasma Arc Welding of
Aluminum Alloys,” Journal of Advanced Metallurgical
Sciences, Vol. 13, No. 1, 2000, pp. 194-200.
[6] S.-C. Chi and L.-C. Hsu, “A Fuzzy Radial Basi s Function
Neural Network for Predicting Multiple Quality Charac-
teristics of Plasma Arc Welding,” Joint 9th IFSA World
Congress and 20th NAFIPS International Conference,
Vancouver, 25-28 July 2001, pp. 2807-2812.
[7] Y. F. Hsiao, Y. S. Tarng and W. J. Huang, “Optimization
of Plasma Arc Welding Parameters by Using the Taguchi
Method with the Grey Relational Analysis,” Journal of
Materials and Manufacturing Processes, Vol. 23, No. 1,
2007, pp. 51-58. doi:10.1080/10426910701524527
[8] K. Siva, N. Muragan and R. Logesh, “Optimization of
Weld Bead Geometry in Plasma Transferred Arc Hard-
facing Austenitic Stainless Steel Plates Using Genetic
Algorithm,” The International Journal of Advanced Manu-
facturing Technology, Vol. 41, No. 1-2, 2008, pp. 24-30.
doi:10.1007/s00170-008-1451-3
[9] A. K. Lakshinarayana, V. Balasubramanian, R. Varaha-
moorthy and S. Babu, “Predicted the Dilution of Plasma
Transferred Arc Hardfacing of Stellite on Carbon Steel
Using Response Surface Methodology,” Metals and Ma-
terials International, Vol. 14, No. 6, 2008, pp. 779-789.
doi:10.3365/met.mat.2008.12.779
[10] V. Balasubramanian, A. K. Lakshminarayanan, R. Vara-
hamoorthy and S. Babu, “Application of Response Sur-
face Methodolody to Prediction of Dilution in Plasma
Transferred Arc Hardfacing of Stainless Steel on Carbon
Steel,” International Journal of Iron and Steel Research,
Vol. 16, No. 1, 2009, pp. 44-53.
[11] E. Taban, A. Dhooge and E. Kaluc, “Plasma Arc Welding
of Modified 12% Cr Stainless Steel,” Materials and Ma-
nufacturing Processes, Vol. 24, No. 6, 2009, pp. 649-656.
doi:10.1080/10426910902769152
[12] N. Kahraman, M. Taskin, B. Gulenc and A. Durgutlu,
“An Investigation into the Effect of Welding Current on
the Plasma Arc Welding of Pure Titanium,” Kovove Ma-
ter, Vol. 48, No. 3, 2010, pp. 179-184.
[13] N. Srimath and N. Muragan, “Prediction and Optimiza-
tion of Weld Bead Geometry of Plasma Transferred Arc
Hardfacing Valve Seat Rings,” European Journal of Sci-
entific Research, Vol. 51, No. 2, 2011, pp. 285-298.
[14] D. C. Montgomery, “ Design and Analysis of Experiments,”
3rd Edition, John Wiley & Sons, New York, 1991.
[15] G. E. P. Box, W. H. Hunter and J. S. Hunter, “Statistics
for Experiments,” John Wiley & Sons, New York, 1978.
[16] J. Ravindra and R. S. Parmar, “Mathematical Model to
Predict Weld Bead Geometry for Flux Cored Arc Weld-
ing,” Journal of Metal Construction, Vol. 19, 1987, pp.
45-52.
[17] W. G. Cochran and G. M. Cox, “Experimental Designs,”
John Wiley & Sons Inc., London, 1957.
[18] T. B. Barker, “Quality by Experimental Design,” ASQC
Quality Press, Milkwakee, 1985.
[19] W. P. Gardiner and G. Gettinby, “Experimental Design
Techniques in Statistical Practice,” Horwood, Chichester,
1998.
[20] K. S. Prasad, Ch. S. Rao and D. N. Rao, “Prediction of
Weld Pool Geometry in Pulsed Current Micro Plasma
Arc Welding of SS304L Stainless Steel Sheets,” Interna-
tional Transaction Journal of Engineering Management
& Applied Sciences & Technologies, Vol. 2, No. 3, 2011,
pp. 325-336.
Copyright © 2012 SciRes. JMMCE